
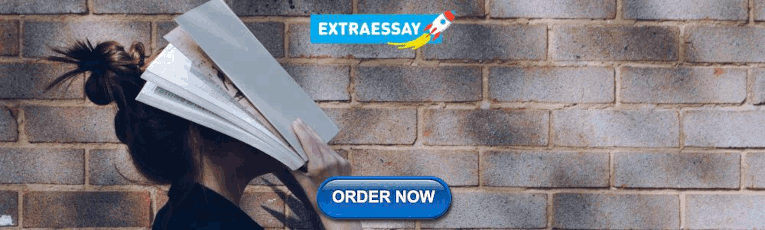
Introduction
Case report, statement of ethics, disclosure statement, funding sources, author contributions, a case of acute kidney injury in a patient with renal hypouricemia without intense exercise.
- Split-Screen
- Article contents
- Figures & tables
- Supplementary Data
- Peer Review
- Open the PDF for in another window
- Get Permissions
- Cite Icon Cite
- Search Site
Daiki Aomura , Kosuke Sonoda , Makoto Harada , Koji Hashimoto , Yuji Kamijo; A Case of Acute Kidney Injury in a Patient with Renal Hypouricemia without Intense Exercise. Case Rep Nephrol Dial 12 May 2020; 10 (1): 26–34. https://doi.org/10.1159/000506673
Download citation file:
- Ris (Zotero)
- Reference Manager
Exercise-induced acute kidney injury (EIAKI) frequently develops in patients with renal hypouricemia (RHUC). However, several cases of RHUC with acute kidney injury (AKI) but without intense exercise have been reported. We encountered a 15-year-old male with RHUC who experienced AKI. He reported no episodes of intense exercise and displayed no other representative risk factors of EIAKI, although a vasopressor had been administered for orthostatic dysregulation before AKI onset. His kidney dysfunction improved with discontinuation of the vasopressor and conservative treatment. Thus, AKI can develop in patients with RHUC in the absence of intense exercise, for which vasopressors may be a risk factor.
Exercise-induced acute kidney injury (EIAKI) is a major complication in patients with renal hypouricemia (RHUC). EIAKI usually develops after intense exercise, such as anaerobic exertion, and is not accompanied by rhabdomyolysis [ 1 ]. However, there are several case reports of patients experiencing EIAKI without intense exercise [ 2-4 ]. Although the pathomechanism and risk factors of EIAKI remain unclear, many reports suggest that an oxidation-reduction imbalance is associated with EIAKI onset [ 5 ]. We herein report a case of acute kidney injury (AKI) in a patient with RHUC in the absence of intense exercise, which may have been caused by an oral vasopressor.
A 15-year-old male complained of strong fatigue after intense exercise since childhood. He had no remarkable medical history apart from allergic rhinitis. After entering high school, he often felt unwell, especially in the morning, and frequently missed classes. He was diagnosed as having orthostatic dysregulation and prescribed amezinium metilsulfate 10 mg/day, but his symptoms persisted. Eight days after the start of treatment he was switched to etilefrine 5 mg/day. However, his fatigue progressively worsened. He was found vomiting and unresponsive after collapsing in the bathroom on the eighth night following the prescription change and taken to the hospital by his family. In the emergency room he exhibited mild consciousness disturbance (Glasgow Coma Scale: E4V4M6) and complained of right lower abdominal pain. Laboratory tests (blood and urine), whole-body computed tomography, and head magnetic resonance imaging did not indicate any abnormalities (serum creatinine level 1.0 mg/dL, uric acid level 7.2 mg/dL). His conscious state and abdominal pain were improved on the next day, but his blood pressure gradually increased from 100/60 to 180/80 mm Hg and his serum creatinine level rose from 1.0 to 5.5 mg/dL during 5 days of admission. He was then transferred to our institution for the treatment of AKI and severe hypertension.
At the time of admission to our hospital the patient was fully conscious and alert. His body temperature was 37.2°C, blood pressure was 161/98 mm Hg, heart rate was 83 beats/min, and respiratory rate was 17 breaths/min. His height was 174 cm and his body weight was 54 kg. Physical examination detected no signs of dehydration, rash, or other abnormalities of the neck, chest, abdomen, or extremities. He had been taking loratadine 10 mg/day for his allergic rhinitis for several months. Both loratadine and etilefrine had been discontinued upon admission to the previous hospital. There was no family history of kidney dysfunction, and he reported no episodes of intense exercise other than daily commuting by bicycle to school. No alcohol consumption, smoking, or illegal drug use were reported. His laboratory data at the time of transfer to our hospital are summarized in Table 1 . Urinalysis showed mild proteinuria (0.66 g/gCr) and elevation of the tubulointerstitial injury marker β2 microglobulin (1,498 μg/L). Hematuria was not observed. His serum level of uric acid was low at 3.2 mg/dL and his fractional excretion of uric acid was high at 49.7%. Laboratory markers of rhabdomyolysis, diabetes mellitus, infection, and collagen diseases such as creatine phosphokinase, hemoglobin A1c, C-reactive protein, and autoimmune antibodies were all within normal range. An electrocardiogram disclosed left anterior hemiblock and nonspecific intraventricular conduction delay that had been detected when he was an elementary school student. A chest X-ray revealed no abnormalities. Ultrasound echography showed bilateral mild kidney swelling with increased renal cortical echogenicity (Fig. 1 ). No stenotic lesions were detected in the aorta or renal arteries, although the resistance index of the intrarenal arteries was slightly high (left 0.69, right 0.69), indicating a circulatory disturbance in the renal microvessels. Hydronephrosis and renal calcification were absent. An ultrasound-guided kidney biopsy performed 3 days after arrival at our hospital showed mild interstitial edema, vascular endothelial cell swelling in the renal interlobular arterioles, and no obvious signs of acute tubular necrosis (ATN) (Fig. 2 ). Treatment with continuous intravenous infusion of extracellular fluids and nicardipine gradually improved his kidney function and hypertension. His serum uric acid level decreased to 1.0 mg/dL (Fig. 3 ), and his fractional excretion of uric acid was at 55.9% at 10 days after admission. He was ultimately diagnosed as having AKI with RHUC and discharged 12 days after transfer to our hospital. Hypouricemia was found in his parents and a sister, indicating a hereditary condition. However, genetic screening did not detect any known causative RHUC mutations on URAT1/SLC22A12 or GLUT9/SLC2A9 .
Main laboratory data on admission to our hospital
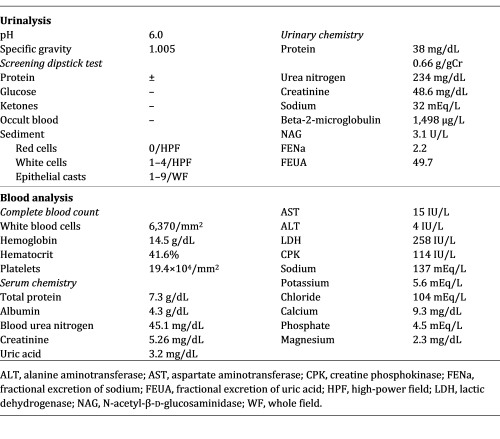
Renal ultrasound showed mild kidney swelling with increased renal cortical echogenicity. Hydronephrosis and renal calcification were not observed. Renal imaging findings were similar bilaterally (left 105 × 62 mm, right 115 × 63 mm).
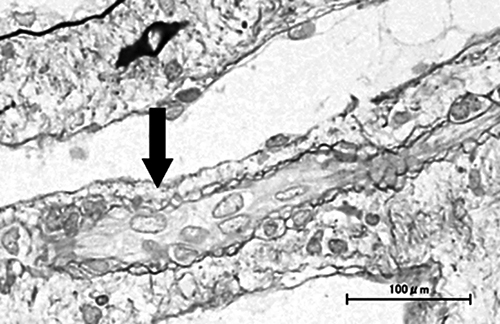
Kidney biopsy specimen findings. Mild interstitial edema and vascular lumen narrowing by endothelial cell swelling (arrow) were detected (periodic acid-methenamine silver stain). No other abnormalities were found, including signs of acute tubular necrosis.
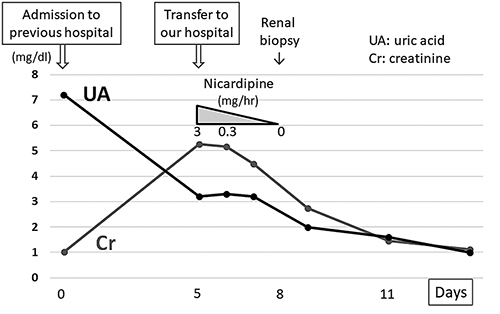
Clinical course of the present case. Vasopressors that had been administered for 15 days were discontinued on admission. After transfer to our hospital, his renal function improved gradually with continuous intravenous infusion of extracellular fluids and nicardipine. The serum uric acid level decreased steadily to 1.0 mg/dL during hospitalization.
Ishikawa et al. [ 6 ] first described EIAKI as AKI with accompanying abdominal or lower back pain after intense exercise, such as a 100-meter dash. EIAKI is differentiated from AKI with rhabdomyolysis by normal or slightly elevated serum myoglobin and creatine phosphokinase levels. EIAKI typically occurs in young males, with more than half having RHUC. Enhanced computed tomography often displays a wedge-shaped contrast defect in the kidneys. As for the clinical course of EIAKI, kidney dysfunction improves naturally without any special treatment [ 1, 7 ]. Although the reported patient had no intense episodes of exercise, EIAKI was diagnosed because he had RHUC, his kidney function recovered naturally, and he was young and male.
Blood pressure and serum creatinine level in our patient increased gradually following admission to the former hospital. As high blood pressure alone might cause AKI, we could not exclude the possible involvement of hypertension in AKI development. However, his serum creatinine level ultimately improved to 0.7 mg/dL after the final discharge despite having been 1.0 mg/dL on first admission, indicating that it had already been elevated by 0.3 mg/dL at the former hospital. Considering the fact that his blood pressure was normal on admission, AKI was thought to have developed before blood pressure elevation. Furthermore, his serum uric acid level was much higher on first admission (7.2 mg/dL) than at discharge (1.0 mg/dL), suggesting AKI onset prior to the former hospital visit. We suspected that AKI caused hypertension, which in turn worsened AKI. The elevation of blood pressure was assumed to be an exacerbation factor of EIAKI rather than its main cause.
The reported patient had no intense episodes of exercise. Lee et al. [ 3 ] described 17 AKI patients with abdominal or lower back pain who exhibited the characteristic patchy kidney sign on enhanced computed tomography. Among them, 5 patients reported no episodes of intense exercise. To the best of our knowledge, there have been 8 patients with EIAKI who did not have any episodes of intense exertion [ 2-4 ], with 5 experiencing infection or analgesic usage before EIAKI onset (Table 2 ), thought to be risk factors of EIAKI in addition to RHUC [ 3, 8, 9 ]. These reports support the notion that EIAKI can develop without intense exercise and the existence of risk factors other than strong exertion. However, to date no reports have focused on the relationship between lack of intense exercise and the etiology and development mechanism of EIAKI.
Clinical findings of current and previous reported cases of EIAKI without strenuous exercise
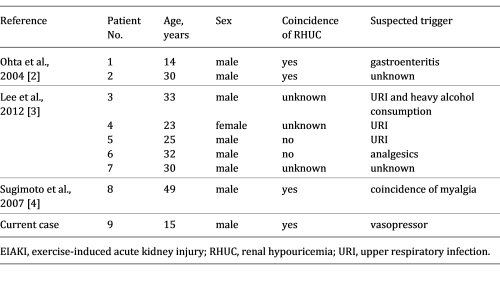
The pathomechanism of EIAKI is unclear, but renal circulatory disturbance by reactive oxygen species (ROS) is thought to be a main cause [ 5 ]. Intense exercise, such as anaerobic exertion, produces large amounts of ROS, which are rapidly removed by uric acid and other scavengers in the healthy population [ 8 ]. Patients with RHUC have insufficient scavengers, resulting in inadequate ROS removal and the subsequent activation of vasoconstrictive factors, vasoconstriction, and renal ischemia [ 2 ]. Since renal vasoconstriction is known to trigger further vasoconstriction and oxidative stress via activation of the renin-angiotensin system and blood pressure elevation [ 10 ], EIAKI patients are thought to show a vicious cycle between oxidative stress and vasoconstriction – oxidative stress causes stronger vasoconstriction and vasoconstriction causes more oxidative stress – culminating in acute and severe renal injury. In the present case, the patient had been taking vasopressors for orthostatic dysregulation for 15 days prior to the onset of AKI. Amezinium metilsulfate inhibits monoamine oxidase activity and suppresses the uptake of noradrenaline, while etilefrine activates type α1 and β1 adrenaline receptors. Thus, both vasopressors increased cardiac output and the constriction of peripheral vessels [ 11, 12 ]. Bellomo et al. [ 13 ] reported that activation of type α1 adrenaline receptors could cause excessive renal vasoconstriction and decreased renal blood flow in models of healthy renal hemodynamics. Radaković et al. [ 14 ] described that adrenaline induction increased ROS and caused a disruption in oxidant/antioxidant balance. Considering these results and the developmental mechanism of EIAKI (i.e., ROS and renal ischemia), we suspect that the vasopressors may have affected the onset or worsening of EIAKI by increasing ROS, exacerbating vasoconstriction, and forming a vicious cycle of diminished renal hemodynamics. Karasawa et al. [ 15 ] reported a case of EIAKI who was given midodrine, another vasopressor, before the onset of EIAKI, and Saito et al. [ 16 ] described that vasoexpansion by low-dose dopamine improved the resistance index of renal arterioles in 2 cases of EIAKI, implying the relation between vasopressors and EIAKI in clinical settings. Although no studies have directly addressed the relationship between vasopressors and EIAKI, past reports and our own results indicate an importance of catecholamine level homeostasis in the pathogenesis of EIAKI. We suspect that vasopressors may be associated with AKI onset in RHUC patients and may be a risk factor of EIAKI.
Renal biopsy showed no significant abnormalities in the present case. Although patients with EIAKI generally exhibit ATN, Ohta et al. [ 2 ] reported no abnormalities in 6 of 28 renal biopsies from EIAKI patients, which implied that EIAKI could develop without ATN. AKI with renal ischemia often causes ATN. However, tubular necrosis is sometimes absent without a sufficient degree or duration of ischemia, and early treatment for renal ischemia leads to a rapid improvement in renal function in such cases [ 17 ]. In the present patient, vasopressors, which might be a risk factor for EIAKI, were discontinued and intravenous antihypertensive medication was induced just after the first admission. The serum uric acid level was temporarily elevated on admission by AKI, and the patient’s scavenging ability with serum uric acid was thought to be temporarily improved. These factors could have mitigated the vicious cycle between renal vasoconstriction and oxidative stress, reduced the severity of renal ischemia, and prevented ATN development. However, as no studies have addressed the cause or meaning of a lack of ATN in some EIAKI patients, a greater number of studies are needed.
In conclusion, AKI can develop in patients with RHUC without intense exercise, possibly through the use of vasopressors. Further related case reports are needed to clarify the association between vasopressor use and AKI in patients with RHUC.
The present case report adhered to the Declaration of Helsinki. Informed consent for publication was obtained from the patient.
The authors declare no conflicts of interest.
The authors received no specific funding for this work.
D. Aomura drafted the article. K. Sonoda, M. Harada, and K. Hashimoto revised the article critically for important intellectual content. Y. Kamijo revised the article critically for important intellectual content and gave final approval of the version to be submitted.

Email alerts
Citing articles via, suggested reading.
- Online ISSN 2296-9705
INFORMATION
- Contact & Support
- Information & Downloads
- Rights & Permissions
- Terms & Conditions
- Catalogue & Pricing
- Policies & Information
- People & Organization
- Stay Up-to-Date
- Regional Offices
- Community Voice
SERVICES FOR
- Researchers
- Healthcare Professionals
- Patients & Supporters
- Health Sciences Industry
- Medical Societies
- Agents & Booksellers
Karger International
- S. Karger AG
- P.O Box, CH-4009 Basel (Switzerland)
- Allschwilerstrasse 10, CH-4055 Basel
- Tel: +41 61 306 11 11
- Fax: +41 61 306 12 34
- Email: [email protected]
- Experience Blog
- Privacy Policy
- Terms of Use
This Feature Is Available To Subscribers Only
Sign In or Create an Account

MAHBOOB RAHMAN, MD, MS, FARIHA SHAD, MD, AND MICHAEL C. SMITH, MD
Am Fam Physician. 2012;86(7):631-639
A more recent article on acute kidney injury is available.
Author disclosure: No relevant financial affiliations to disclose.
Acute kidney injury is characterized by abrupt deterioration in kidney function, manifested by an increase in serum creatinine level with or without reduced urine output. The spectrum of injury ranges from mild to advanced, sometimes requiring renal replacement therapy. The diagnostic evaluation can be used to classify acute kidney injury as prerenal, intrinsic renal, or postrenal. The initial workup includes a patient history to identify the use of nephrotoxic medications or systemic illnesses that might cause poor renal perfusion or directly impair renal function. Physical examination should assess intravascular volume status and identify skin rashes indicative of systemic illness. The initial laboratory evaluation should include measurement of serum creatinine level, complete blood count, urinalysis, and fractional excretion of sodium. Ultrasonography of the kidneys should be performed in most patients, particularly in older men, to rule out obstruction. Management of acute kidney injury involves fluid resuscitation, avoidance of nephrotoxic medications and contrast media exposure, and correction of electrolyte imbalances. Renal replacement therapy (dialysis) is indicated for refractory hyperkalemia; volume overload; intractable acidosis; uremic encephalopathy, pericarditis, or pleuritis; and removal of certain toxins. Recognition of risk factors (e.g., older age, sepsis, hypovolemia/shock, cardiac surgery, infusion of contrast agents, diabetes mellitus, preexisting chronic kidney disease, cardiac failure, liver failure) is important. Team-based approaches for prevention, early diagnosis, and aggressive management are critical for improving outcomes.
The incidence of acute kidney injury has increased in recent years, both in the community and in hospital settings. 1 , 2 The estimated incidence of acute kidney injury is two to three cases per 1,000 persons. 3 Seven percent of hospitalized patients and about two-thirds of patients in intensive care units develop acute kidney injury, 2 often as part of the multiple organ dysfunction syndrome. 4
Acute kidney injury is associated with a high rate of adverse outcomes; mortality rates range between 25 and 80 percent, depending on the cause and the clinical status of the patient. 5 – 7 These data highlight the importance of recognition and appropriate management, usually in collaboration with nephrologists and other subspecialists.
Acute kidney injury is defined as an abrupt (within 48 hours) reduction in kidney function based on an elevation in serum creatinine level, a reduction in urine output, the need for renal replacement therapy (dialysis), or a combination of these factors. It is classified in three stages ( Table 1 ) . 8 The term acute kidney injury should replace terms such as acute renal failure and acute renal insufficiency, which previously have been used to describe the same clinical condition.
The causes of acute kidney injury can be divided into three categories ( Table 2 9 ) : prerenal (caused by decreased renal perfusion, often because of volume depletion), intrinsic renal (caused by a process within the kidneys), and postrenal (caused by inadequate drainage of urine distal to the kidneys). In patients who already have underlying chronic kidney disease, any of these factors, but especially volume depletion, may cause acute kidney injury in addition to the chronic impairment of renal function.
PRERENAL CAUSES
Approximately 70 percent of community-acquired cases of acute kidney injury are attributed to prerenal causes. 10 In these cases, underlying kidney function may be normal, but decreased renal perfusion associated with intravascular volume depletion (e.g., from vomiting or diarrhea) or decreased arterial pressure (e.g., from heart failure or sepsis) results in a reduced glomerular filtration rate. Autoregulatory mechanisms often can compensate for some degree of reduced renal perfusion in an attempt to maintain the glomerular filtration rate. In patients with preexisting chronic kidney disease, however, these mechanisms are impaired, and the susceptibility to develop acute-on-chronic renal failure is higher. 11
Several medications can cause prerenal acute kidney injury. Notably, angiotensin-converting enzyme inhibitors and angiotensin receptor blockers can impair renal perfusion by causing dilation of the efferent arteriole and reduce intraglomerular pressure. Nonsteroidal anti-inflammatory drugs also can decrease the glomerular filtration rate by changing the balance of vasodilatory/vasoconstrictive agents in the renal microcirculation. These drugs and others limit the normal homeostatic responses to volume depletion and can be associated with a decline in renal function. In patients with prerenal acute kidney injury, kidney function typically returns to baseline after adequate volume status is established, the underlying cause is treated, or the offending drug is discontinued.
INTRINSIC RENAL CAUSES
Intrinsic renal causes are also important sources of acute kidney injury and can be categorized by the component of the kidney that is primarily affected (i.e., tubular, glomerular, interstitial, or vascular).
Acute tubular necrosis is the most common type of intrinsic acute kidney injury in hospitalized patients. The cause is usually ischemic (from prolonged hypotension) or nephrotoxic (from an agent that is toxic to the tubular cells). In contrast to a prerenal etiology, acute kidney injury caused by acute tubular necrosis does not improve with adequate repletion of intravascular volume and blood flow to the kidneys. Both ischemic and nephrotoxic acute tubular necrosis can resolve over time, although temporary renal replacement therapy may be required, depending on the degree of renal injury and the presence of preexisting chronic kidney disease.
Glomerular causes of acute kidney injury are the result of acute inflammation of blood vessels and glomeruli. Glomerulonephritis is usually a manifestation of a systemic illness (e.g., systemic lupus erythematosus) or pulmonary renal syndromes (e.g., Goodpasture syndrome, Wegener granulomatosis). History, physical examination, and urinalysis are crucial for diagnosing glomerulonephritis ( Table 3 9 and Figure 1 12 ) . Because management often involves administration of immunosuppressive or cytotoxic medications with potentially severe adverse effects, renal biopsy is often required to confirm the diagnosis before initiating therapy.
Acute interstitial nephritis can be secondary to many conditions, but most cases are related to medication use, making patient history the key to diagnosis. In about one-third of cases, there is a history of maculopapular erythematous rash, fever, arthralgias, or a combination of these symptoms. 13 Eosinophiluria may be found in patients with acute interstitial nephritis, but it is not pathognomonic of this disease. A kidney biopsy may be needed to distinguish between allergic interstitial nephritis and other renal causes of acute kidney injury. In addition to discontinuing offending agents, steroids may be beneficial if given early in the course of disease. 14
Acute events involving renal arteries or veins can also lead to intrinsic acute kidney injury. Renal atheroembolic disease is the most common cause and is suspected with a recent history of arterial catheterization, the presence of a condition requiring anticoagulation, or after vascular surgery. Physical examination and history provide important clues to the diagnosis ( Table 3 9 ) . Vascular causes of acute kidney injury usually require imaging to confirm the diagnosis.
POSTRENAL CAUSES
Postrenal causes typically result from obstruction of urinary flow, and prostatic hypertrophy is the most common cause of obstruction in older men. Prompt diagnosis followed by early relief of obstruction is associated with improvement in renal function in most patients.
Clinical Presentation
Clinical presentation varies with the cause and severity of renal injury, and associated diseases. Most patients with mild to moderate acute kidney injury are asymptomatic and are identified on laboratory testing. Patients with severe cases, however, may be symptomatic and present with listlessness, confusion, fatigue, anorexia, nausea, vomiting, weight gain, or edema. 15 Patients can also present with oliguria (urine output less than 400 mL per day), anuria (urine output less than 100 mL per day), or normal volumes of urine (nonoliguric acute kidney injury). Other presentations of acute kidney injury may include development of uremic encephalopathy (manifested by a decline in mental status, asterixis, or other neurologic symptoms), anemia, or bleeding caused by uremic platelet dysfunction.
A patient history and physical examination, with an emphasis on assessing the patient’s volume status, are crucial for determining the cause of acute kidney injury ( Table 3 9 ) . The history should identify use of nephrotoxic medications or systemic illnesses that might cause poor renal perfusion or directly impair renal function. Physical examination should assess intravascular volume status and any skin rashes indicative of systemic illness. The initial laboratory evaluation should include urinalysis, complete blood count, and measurement of serum creatinine level and fractional excretion of sodium (FE Na ). Imaging studies can help rule out obstruction. Useful tests are summarized in Table 4 . 16 Figure 1 presents an overview of the diagnosis and management of acute kidney injury. 12
SERUM CREATININE LEVEL
It is important to compare the patient’s current serum creatinine level with previous levels to determine the duration and acuity of the disease. The definition of acute kidney injury indicates that a rise in creatinine has occurred within 48 hours, although in the outpatient setting, it may be hard to ascertain when the rise actually happened. A high serum creatinine level in a patient with a previously normal documented level suggests an acute process, whereas a rise over weeks to months represents a subacute or chronic process.
Urinalysis is the most important noninvasive test in the initial workup of acute kidney injury. Findings on urinalysis guide the differential diagnosis and direct further workup ( Figure 1 12 ) .
COMPLETE BLOOD COUNT
The presence of acute hemolytic anemia with the peripheral smear showing schistocytes in the setting of acute kidney injury should raise the possibility of hemolytic uremic syndrome or thrombotic thrombocytopenic purpura.
URINE ELECTROLYTES
In patients with oliguria, measurement of FE Na is helpful in distinguishing prerenal from intrinsic renal causes of acute kidney injury. FE Na is defined by the following formula:
Online calculators are also available. A value less than 1 percent indicates a prerenal cause of acute kidney injury, whereas a value greater than 2 percent indicates an intrinsic renal cause. In patients on diuretic therapy, however, a FE Na higher than 1 percent may be caused by natriuresis induced by the diuretic, and is a less reliable measure of a prerenal state. In such cases, fractional excretion of urea may be helpful, with values less than 35 percent indicating a prerenal cause. FE Na values less than 1 percent are not specific for prerenal causes of acute kidney injury because these values can occur in other conditions, such as contrast nephropathy, rhabdomyolysis, acute glomerulonephritis, and urinary tract obstruction.
IMAGING STUDIES
Renal ultrasonography should be performed in most patients with acute kidney injury, particularly in older men, to rule out obstruction (i.e., a postrenal cause). 17 , 18 The presence of postvoid residual urine greater than 100 mL (determined by a bladder scan or via urethral catheterization if bladder scan is unavailable) suggests postrenal acute kidney injury and requires renal ultrasonography to detect hydronephrosis or outlet obstruction. To diagnose extrarenal causes of obstruction (e.g., pelvic tumors), other imaging modalities, such as computed tomography or magnetic resonance imaging, may be required.
RENAL BIOPSY
Renal biopsy is reserved for patients in whom prerenal and postrenal causes of acute kidney injury have been excluded and the cause of intrinsic renal injury is unclear. Renal biopsy is particularly important when clinical assessment and laboratory investigations suggest a diagnosis that requires confirmation before disease-specific therapy (e.g., immunosuppressive medications) is instituted. Renal biopsy may need to be performed urgently in patients with oliguria who have rapidly worsening acute kidney injury, hematuria, and red blood cell casts. In this setting, in addition to indicating a diagnosis that requires immunosuppressive therapy, the biopsy may support the initiation of special therapies, such as plasmapheresis if Goodpasture syndrome is present.
Optimal management of acute kidney injury requires close collaboration among primary care physicians, nephrologists, hospitalists, and other subspecialists participating in the care of the patient. After acute kidney injury is established, management is primarily supportive.
Patients with acute kidney injury generally should be hospitalized unless the condition is mild and clearly resulting from an easily reversible cause. The key to management is assuring adequate renal perfusion by achieving and maintaining hemodynamic stability and avoiding hypovolemia. In some patients, clinical assessment of intravascular volume status and avoidance of volume overload may be difficult, in which case measurement of central venous pressures in an intensive care setting may be helpful.
If fluid resuscitation is required because of intravascular volume depletion, isotonic solutions (e.g., normal saline) are preferred over hyperoncotic solutions (e.g., dextrans, hydroxyethyl starch, albumin). 19 A reasonable goal is a mean arterial pressure greater than 65 mm Hg, which may require the use of vasopressors in patients with persistent hypotension. 20 Renal-dose dopamine is associated with poorer outcomes in patients with acute kidney injury; it is no longer recommended. 21 Cardiac function can be optimized as needed with positive inotropes, or afterload and preload reduction.
Attention to electrolyte imbalances (e.g., hyperkalemia, hyperphosphatemia, hypermagnesemia, hyponatremia, hypernatremia, metabolic acidosis) is important. Severe hyperkalemia is defined as potassium levels of 6.5 mEq per L (6.5 mmol per L) or greater, or less than 6.5 mEq per L with electrocardiographic changes typical of hyperkalemia (e.g., tall, peaked T waves). In severe hyperkalemia, 5 to 10 units of regular insulin and dextrose 50% given intravenously can shift potassium out of circulation and into the cells. Calcium gluconate (10 mL of 10% solution infused intravenously over five minutes) is also used to stabilize the membrane and reduce the risk of arrhythmias when there are electrocardiographic changes showing hyperkalemia. In patients without electrocardiographic evidence of hyperkalemia, calcium gluconate is not necessary, but sodium polystyrene sulfonate (Kayexalate) can be given to lower potassium levels gradually, and loop diuretics can be used in patients who are responsive to diuretics. Dietary intake of potassium should be restricted.
The main indication for use of diuretics is management of volume overload. Intravenous loop diuretics, as a bolus or continuous infusion, can be helpful for this purpose. However, it is important to note that diuretics do not improve morbidity, mortality, or renal outcomes, and should not be used to prevent or treat acute kidney injury in the absence of volume overload. 22
All medications that may potentially affect renal function by direct toxicity or by hemodynamic mechanisms should be discontinued, if possible. For example, metformin (Glucophage) should not be given to patients with diabetes mellitus who develop acute kidney injury. The dosages of essential medications should be adjusted for the lower level of kidney function. Avoidance of iodinated contrast media and gadolinium is important and, if imaging is needed, noncontrast studies are recommended.
Supportive therapies (e.g., antibiotics, maintenance of adequate nutrition, mechanical ventilation, glycemic control, anemia management) should be pursued based on standard management practices. In patients with rapidly progressive glomerulonephritis, treatment with pulse steroids, cytotoxic therapy, or a combination may be considered, often after confirmation of the diagnosis by kidney biopsy. 23 In some patients, the metabolic consequences of acute kidney injury cannot be adequately controlled with conservative management, and renal replacement therapy will be required. The indications for initiation of renal replacement therapy include refractory hyperkalemia, volume overload refractory to medical management, uremic pericarditis or pleuritis, uremic encephalopathy, intractable acidosis, and certain poisonings and intoxications (e.g., ethylene glycol, lithium). 24
Patients with acute kidney injury are more likely to develop chronic kidney disease in the future. They are also at higher risk of end-stage renal disease and premature death. 25 – 27 Patients who have an episode of acute kidney injury should be monitored for the development or worsening of chronic kidney disease.
Because of the morbidity and mortality associated with acute kidney injury, it is important for primary care physicians to identify patients who are at high risk of developing this type of injury and to implement preventive strategies. Those at highest risk include adults older than 75 years; persons with diabetes or preexisting chronic kidney disease; persons with medical problems such as cardiac failure, liver failure, or sepsis; and those who are exposed to contrast agents or who are undergoing cardiac surgery. 28 Preventive strategies can be tailored to the clinical circumstances of the individual patient ( Table 5 ) . 19 – 21 , 27 , 29 – 31
Data Sources: We searched PubMed (also with the Clinical Queries function), the Cochrane Database of Systematic Reviews, and the National Guidelines Clearinghouse using the key words AKI, acute kidney injury, and acute renal failure. Search date: February 2012.
Hsu CY, McCulloch CE, Fan D, Ordoñez JD, Chertow GM, Go AS. Community-based incidence of acute renal failure. Kidney Int. 2007;72(2):208-212.
Nash K, Hafeez A, Hou S. Hospital-acquired renal insufficiency. Am J Kidney Dis. 2002;39(5):930-936.
Hoste EA, Schurgers M. Epidemiology of acute kidney injury: how big is the problem?. Crit Care Med. 2008;36(4 suppl):S146-S151.
Hoste EA, Clermont G, Kersten A, et al. RIFLE criteria for acute kidney injury are associated with hospital mortality in critically ill patients: a cohort analysis. Crit Care. 2006;10(3):R73.
Ympa YP, Sakr Y, Reinhart K, Vincent JL. Has mortality from acute renal failure decreased? A systematic review of the literature. Am J Med. 2005;118(8):827-832.
Gruberg L, Weissman NJ, Pichard AD, et al. Impact of renal function on morbidity and mortality after percutaneous aortocoronary saphenous vein graft intervention. Am Heart J. 2003;145(3):529-534.
Uchino S, Kellum JA, Bellomo R, et al.; Beginning and Ending Supportive Therapy for the Kidney (BEST Kidney) Investigators. Acute renal failure in critically ill patients: a multinational, multicenter study. JAMA. 2005;294(7):813-818.
Mehta RL, Kellum JA, Shah SV, et al. Acute Kidney Injury Network: report of an initiative to improve outcomes in acute kidney injury. Crit Care. 2007;11(2):R31.
Holley JL. Clinical approach to the diagnosis of acute renal failure. In: Greenberg A, Cheung AK, eds. Primer on Kidney Diseases . 5th ed. Philadelphia, Pa.: National Kidney Foundation; 2009.
Kaufman J, Dhakal M, Patel B, Hamburger R. Community-acquired acute renal failure. Am J Kidney Dis. 1991;17(2):191-198.
Christensen PK, Hansen HP, Parving HH. Impaired autoregulation of GFR in hypertensive non-insulin dependent diabetic patients. Kidney Int. 1997;52(5):1369-1374.
Smith MC. Acute renal failure. In: Resnick MI, Elder JS, Spirnak JP, eds. Clinical Decisions in Urology . 3rd ed. Hamilton, Ontario, Canada: BC Decker, Inc.; 2004:60–63.
Clarkson MR, Giblin L, O’Connell FP, et al. Acute interstitial nephritis: clinical features and response to corticosteroid therapy. Nephrol Dial Transplant. 2004;19(11):2778-2783.
González E, Gutiérrez E, Galeano C, et al.; Grupo Madrileño De Nefritis Intersticiales. Early steroid treatment improves the recovery of renal function in patients with drug-induced acute interstitial nephritis. Kidney Int. 2008;73(8):940-946.
Meyer TW, Hostetter TH. Uremia. N Engl J Med. 2007;357(13):1316-1325.
Agrawal M, Swartz R. Acute renal failure [published correction appears in Am Fam Physician . 2001;63(3):445]. Am Fam Physician. 2000;61(7):2077-2088.
Lewington A, Kanagasundaram S. Clinical practice guidelines: acute kidney injury. 2011. http://www.renal.org/clinical/guidelinessection/AcuteKidneyInjury.aspx . Accessed September 7, 2012.
O’Neill WC. Sonographic evaluation of renal failure. Am J Kidney Dis. 2000;35(6):1021-1038.
Schortgen F, Lacherade JC, Bruneel F, et al. Effects of hydroxyethylstarch and gelatin on renal function in severe sepsis: a multicentre randomised study. Lancet. 2001;357(9260):911-916.
Brochard L, Abroug F, Brenner M, et al. An Official ATS/ERS/ESICM/SCCM/SRLF Statement: Prevention and Management of Acute Renal Failure in the ICU Patient: an international consensus conference in intensive care medicine. Am J Respir Crit Care Med. 2010;181(10):1128-1155.
Friedrich JO, Adhikari N, Herridge MS, Beyene J. Meta-analysis: low-dose dopamine increases urine output but does not prevent renal dysfunction or death. Ann Intern Med. 2005;142(7):510-524.
Ho KM, Sheridan DJ. Meta-analysis of frusemide to prevent or treat acute renal failure. BMJ. 2006;333(7565):420.
Walters G, Willis NS, Craig JC. Interventions for renal vasculitis in adults. Cochrane Database Syst Rev. ;2008(3):CD003232.
Mehta RL. Indications for dialysis in the ICU: renal replacement vs. renal support. Blood Purif. 2001;19(2):227-232.
Goldberg R, Dennen P. Long-term outcomes of acute kidney injury. Adv Chronic Kidney Dis. 2008;15(3):297-307.
Coca SG, Yusuf B, Shlipak MG, Garg AX, Parikh CR. Long-term risk of mortality and other adverse outcomes after acute kidney injury: a systematic review and meta-analysis. Am J Kidney Dis. 2009;53(6):961-973.
Pession A, Masetti R, Gaidano G, et al. Risk evaluation, prophylaxis, and treatment of tumor lysis syndrome: consensus of an Italian expert panel. Adv Ther. 2011;28(8):684-697.
Leblanc M, Kellum JA, Gibney RT, Lieberthal W, Tumlin J, Mehta R. Risk factors for acute renal failure: inherent and modifiable risks. Curr Opin Crit Care. 2005;11(6):533-536.
Rundback JH, Nahl D, Yoo V. Contrast-induced nephropathy. J Vasc Surg. 2011;54(2):575-579.
Nadim MK, Kellum JA, Davenport A, et al. Hepatorenal syndrome: the 8th international consensus conference of the Acute Dialysis Quality Initiative (ADQI) group. Crit Care. 2012;16(1):R23.
Auron M, Harte B, Kumar A, Michota F. Renin-angiotensin system antagonists in the perioperative setting: clinical consequences and recommendations for practice. Postgrad Med J. 2011;87(1029):472-481.
Continue Reading
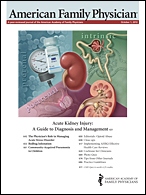
More in AFP
More in pubmed.
Copyright © 2012 by the American Academy of Family Physicians.
This content is owned by the AAFP. A person viewing it online may make one printout of the material and may use that printout only for his or her personal, non-commercial reference. This material may not otherwise be downloaded, copied, printed, stored, transmitted or reproduced in any medium, whether now known or later invented, except as authorized in writing by the AAFP. See permissions for copyright questions and/or permission requests.
Copyright © 2024 American Academy of Family Physicians. All Rights Reserved.
Acute Kidney Injury Case Study (60 min)
Watch More! Unlock the full videos with a FREE trial
Included In This Lesson
Study tools.
Access More! View the full outline and transcript with a FREE trial
Case Study Objectives
- Analyze and interpret clinical data and patient assessments to identify signs and symptoms of acute kidney injury (AKI) in a real-life patient scenario.
- Apply critical thinking skills to recognize the physiological mechanisms contributing to the development of AKI, considering factors such as dehydration, contrast dye exposure, and prolonged NPO status.
- Evaluate the appropriate nursing actions and interventions required at various stages of AKI management, including fluid resuscitation, diuretic therapy, and ongoing assessment.
- Anticipate and suggest potential preventive measures for AKI, emphasizing the importance of pre- and post-contrast scan IV fluid administration in vulnerable patients.
- Understand the significance of monitoring laboratory values, such as BUN, creatinine, GFR, and electrolytes, to assess kidney function and guide treatment decisions in AKI cases.
By actively engaging with this acute kidney injury case study, nursing students will enhance their clinical reasoning skills and gain valuable insights into the assessment, management, and prevention of AKI in real-world healthcare scenarios.
Kidney Injury Case Study
Ms. Barkley is a thin, frail 64-year-old female presenting from a nursing home for acute abdominal pain, nausea, and vomiting x 2 days. She receives a CT scan with IV contrast. Findings show no acute bleeding, but a possible small bowel obstruction. She is admitted for bowel rest, with the following written orders from the provider:
- Continuous Telemetry
- Strict I&O measurements
- Keep SpO 2 > 92%
- Keep NPO (strict)
- Hydrocodone/Acetaminophen 5-325 mg PO q6h PRN moderate to severe pain
- Ondansetron 4mg PRN nausea
She is admitted to the unit at the beginning of shift, and the UAP reports the following vital signs: HR 103 RR 16 BP 118/68 SpO 2 96% Pain 6/10
Which order would you question or request clarification for? Why?
- The Ondansetron order is incomplete. There is no route or frequency ordered
What additional nursing assessments need to be performed?
- Assess abdomen – inspect, auscultate, palpate and percuss. Assess for tenderness over specific areas, feel for masses, and look for guarding.
- Listen to heart and lung sounds to ensure no cardiac involvement
- Assess pain with a detailed pain assessment so that pain can be treated appropriately
- Assess skin – the patient has had nausea/vomiting for 2 days, there may be some dehydration – check for tenting.
At the end of the 12-hour shift, vital signs are as follows: HR 96 RR 22
BP 147/80 SpO 2 93%
The nurse recognizes that the patient has not voided all day and assists the patient to the bathroom. The patient voids 200 mL dark, concentrated urine.
What nursing action(s) should be implemented at this time? Who should this information be passed on to?
- Document the output, notify the provider of the decreased urine output
- This information needs to be passed onto the oncoming nurse so that he or she can closely monitor the patient’s urine output.
What diagnostic tests would you expect the provider to order? Why?
- Expect an order for a Basic Metabolic Panel or a Renal Function panel
- It seems like her kidneys aren’t making urine as they should, or she may be severely dehydrated. A chemistry panel can tell us more information about the source of decreased urine output.
Provider orders a 500 mL bolus of Normal Saline (0.9%) IV over 1 hour and a renal function panel, which is drawn promptly by the nurse. After 6 hours, Ms. Barkley still has had no further urine output. A bladder scan shows approximately 60 mL of urine in the bladder. A head-to-toe assessment now reveals crackles in Ms. Barkley’s lungs and her SpO 2 is 89%
The renal function panel has resulted: BUN 56 mg/dL Na 132 mg/dL Cr 3.6 mg/dL Ca 7.7 mg/dL GFR 47 mL/min/m 2 Phos 4.8 mg/dL K 5.5 mEq/L Mg 1.4 mg/dL
What nursing action(s) should be implemented at this time?
- Administer O2 2 lpm via nasal cannula (to keep sats > 92%)
- Notify provider of lab results, especially BUN/Cr, GFR, and Potassium – as these indicate there is kidney involvement.
What orders should be anticipated from the provider?
- The patient may need more fluids, she’s been vomiting for 2 days and NPO for another 12 hours with no IV fluids.
- The patient may require diuretics to remove the excess fluid from her lungs and to determine the level of function of her kidneys
What is going on physiologically with Ms. Barkley at this time? Explain what contributed to the development of this condition
- Ms. Barkley seems to have developed an acute kidney injury or acute kidney failure.
- The likely contributors are the severe dehydration coupled with the IV contrast and 12+ hours of being NPO and having no IV fluids. This caused a low-flow state to the kidneys (pre-renal) as well as possible damage to the kidneys themselves because of the contrast (intra-renal).
The provider orders to give 1L bolus of Normal Saline (0.9%) over 1 hour, then 125 mL/hr of Normal Saline continuously. The provider also orders a one-time dose of 40 mg Furosemide IV push and to re-check the Renal Function Panel in 6 hours. Ms. Barkley diuresis approximately 600 mL in 2 hours and her lungs now sound clear to auscultation.
Over the next two days, Ms. Barkley’s hourly urine output begins to improve and her BUN, Creatinine, and GFR return to normal ranges. Her small bowel obstruction resolves on its own and she is able to begin taking PO food and fluids.
What could have been done, if anything, to prevent Acute Kidney Injury for Ms. Barkley?
- The best option would have been to give Ms. Barkley IV fluids before and after her contrast scan, and to make sure she had maintenance IV fluids infusing while she was NPO.
- Depending on the patient’s kidney function, it isn’t always preventable, but in this case, it seems there was more that could have been done.
View the FULL Outline
When you start a FREE trial you gain access to the full outline as well as:
- SIMCLEX (NCLEX Simulator)
- 6,500+ Practice NCLEX Questions
- 2,000+ HD Videos
- 300+ Nursing Cheatsheets
“Would suggest to all nursing students . . . Guaranteed to ease the stress!”
Nursing Case Studies

This nursing case study course is designed to help nursing students build critical thinking. Each case study was written by experienced nurses with first hand knowledge of the “real-world” disease process. To help you increase your nursing clinical judgement (critical thinking), each unfolding nursing case study includes answers laid out by Blooms Taxonomy to help you see that you are progressing to clinical analysis.We encourage you to read the case study and really through the “critical thinking checks” as this is where the real learning occurs. If you get tripped up by a specific question, no worries, just dig into an associated lesson on the topic and reinforce your understanding. In the end, that is what nursing case studies are all about – growing in your clinical judgement.
Nursing Case Studies Introduction
Cardiac nursing case studies.
- 6 Questions
- 7 Questions
- 5 Questions
- 4 Questions
GI/GU Nursing Case Studies
- 2 Questions
- 8 Questions
Obstetrics Nursing Case Studies
Respiratory nursing case studies.
- 10 Questions
Pediatrics Nursing Case Studies
- 3 Questions
- 12 Questions
Neuro Nursing Case Studies
Mental health nursing case studies.
- 9 Questions
Metabolic/Endocrine Nursing Case Studies
Other nursing case studies.
Acute Kidney Injury and Chronic Kidney Disease and Their Impacts on Prognosis among Patients with Severe COVID-19 Pneumonia: An Expert Center Case-Cohort Study
Affiliations.
- 1 Department of Anesthesiology and Intensive Care, COVID-19 Hospital, Military Institute of Medicine-National Research Institute, Szaserów 128 Str., 04-141 Warsaw, Poland.
- 2 Department of Nephrology, Internal Diseases and Dialysis, COVID-19 Hospital, Military Institute of Medicine-National Research Institute, Szaserów 128 Str., 04-141 Warsaw, Poland.
- PMID: 38592301
- PMCID: PMC10932456
- DOI: 10.3390/jcm13051486
Background : Acute kidney injury (AKI) is associated with substantial mortality. In this case-control study, we analyzed the impacts of AKI and chronic kidney disease (CKD) on outcomes in a group of 323 patients with severe COVID-19. The correlation of clinical and laboratory data with AKI and CKD was also analyzed. Methods: A retrospective case-control study was conducted among AKI, CKD, and normal kidney function (NKF) groups hospitalized in a COVID-19 center in 2021. Results: AKI patients had higher in-hospital mortality (55.2 vs. 18.8%, p < 0.001), more frequent transfers from the HDU to ICU (57.5 vs. 12.9%, p < 0.001), and prolonged hospital stays (15.4 ± 10.7 vs. 10.7 ± 6.7 days, p < 0.001) compared to the NKF group. AKI was a predictor of death (OR 4.794, 95%CI: 2.906-7.906, p < 0.001). AKI patients also had broader lung parenchymal involvement and higher inflammatory markers compared to the NKF group. Patients with prior CKD had higher in-hospital mortality compared to the NKF group (64.0 vs. 18.8%, p < 0.001, OR 4.044, 95%CI: 1.723-9.490, p = 0.013); however, transfers from the HDU to ICU were not more frequent (16.0 vs. 12.9%, p = 0.753). Conclusions : AKI among COVID-19 patients was correlated with more ICU transfers, higher morbidity, and greater markers of severe disease. Patients with CKD had a higher mortality; however, the rate of ICU transfer was not substantially higher due to their poor prognosis.
Keywords: COVID-19; acute kidney injury; mortality; pneumonia; risk factors.
Grants and funding
Advertisement

- Previous Issue
- Previous Article
- Next Article
Case Report
Case scenario: hemodynamic management of postoperative acute kidney injury.
Received from the Department of Anesthesiology and Critical Care, Lariboisière Hospital, Assistance Publique-Hopitaux de Paris; University of Paris 7 Denis Diderot, Paris, France. Submitted for publication September 23, 2012. Accepted for publication January 29, 2013. Funding was received from the Ministère de la Recherche, Paris, France, plan quadriennal EA3509. Figures 1–4 were drawn by Annemarie B. Johnson, C.M.I., Medical Illustrator, Wake Forest University School of Medicine Creative Communications, Wake Forest University Medical Center, Winston-Salem, North Carolina.
- Split-Screen
- Article contents
- Figures & tables
- Supplementary Data
- Peer Review
- Open the PDF for in another window
- Cite Icon Cite
- Get Permissions
- Search Site
Matthieu Legrand , Didier Payen; Case Scenario: Hemodynamic Management of Postoperative Acute Kidney Injury. Anesthesiology 2013; 118:1446–1454 doi: https://doi.org/10.1097/ALN.0b013e3182923e8a
Download citation file:
- Ris (Zotero)
- Reference Manager
Acute kidney injury (AKI) is associated with poor outcome both in critically ill patients and after major surgery. 1 The occurrence of AKI has been associated with poor short-term and long-term outcome, increased risk of chronic renal failure, and increased risk of death. 2 Several risk factors of postoperative AKI have been identified, and may help identifying patients with the highest risk of AKI. However, recognizing contributors to AKI ( e.g. , systemic inflammation, systemic hemodynamics alterations, nephrotoxic agents, and others) remains a challenge for anesthesiologists and intensivists because these factors are often associated and AKI multifactorial.
The early diagnosis of AKI remains another issue. Interest in the development and validation of AKI biomarkers has increased among the medical community. In this article, we analyze the risk factors of and contributors to AKI after major surgery, and specifically discuss the strategy of fluid management and potential negative outcome associated with inappropriate fluid administration, with a case scenario intended to illustrate the current knowledge of perioperative AKI. We emphasize hemodynamic management for the prevention and correction of acute renal failure.
A 59-yr-old woman with a history of diabetes and hypertension underwent abdominal surgery for recurrent ovarian cancer. She had received systemic chemotherapy during the 18 months preceding the surgery, including paclitaxel, carboplatin, bevacizumab, doxorubicin, and cyclophosphamide, and had remained asymptomatic since then. The surgery included an ovarian resection and peritoneal carcinosis cytoreduction. The only preoperative medication was an angiotensin-converting enzyme inhibitor to treat arterial hypertension. The preoperative creatinine clearance was estimated at 80ml/min (Modification of Diet in Renal Disease formula). Because she was asymptomatic (no dyspnea or recent change in her clinical status), left ventricular function was not preoperatively assessed.
The known large fluid losses associated with peritoneal carcinosis cytoreduction, intraoperative oliguria, and hypotension led to the infusion of a total of 24 ml·kg −1 ·h −1 of crystalloids during the 9-h surgery (half saline and half Ringer’s lactate solutions). Perioperative maintenance of mean arterial pressure at 70 mmHg was achieved by intravenous infusion of neosynephrine (0.35 μg·kg −1 ·min −1 ). In the recovery room, cold extremities and discrete knee mottles were noted, which motivated a switch to norepinephrine infusion (0.2–0.3 μg·kg −1 ·min −1 ). Because of oliguria during the surgical procedure and anuria in the immediate postoperative period, with urine output less than 0.5 ml·kg −1 ·h −1 , the patient was transferred to the postoperative intensive care unit (ICU). Blood analysis showed a metabolic acidosis, with a chloride concentration of 114 m m and bicarbonates of 12 m m , with a normal anion gap (14 m m ). Serum alanine aminotransferase and alanine transaminase were increased (245 and 257U, respectively), and serum troponin T was 0.223 μg/l. ICU-admission urine level of neutrophil gelatinase-associated lipocalin was 353 ng/mmol urine creatinine. Serum cystatine C was 1mg/l, urine -1 microglobulin was 90mg/l, and the fractional excretion of urea was 29%.
At admission, central venous pressure (CVP) was measured at 24 mmHg, with central venous oxygen saturation (ScvO 2 ) at 66%. Transthoracic echocardiography revealed a severe left ventricular dysfunction, with an ejection fraction of 25%, global hypokinesia, right ventricular dilation, systolic pulmonary arterial pressure at 30 mmHg, and low cardiac output (2 l/min). The serum level of brain natriuretic peptide was 1244 ng/ml.
Norepinephrine was switched to epinephrine, which led to an increase in cardiac output to 4.5 l/min and ScvO2 to 88% and a CVP decrease to 15 mmHg. Intravenous infusion of furosemide was initiated, which increased urine output. AKI was reversed within 72h and epinephrine was stopped 24h later. The patient was discharged from the ICU after 5 days. Serum creatinine at hospital discharge was 60 μ m . A cardiac magnetic resonance imaging performed 2 months later showed global hypokinesia (left ventricular ejection fraction 21%) with no sign of hypoperfusion. The final diagnosis was acute decompensated heart failure due to chemotherapy toxicity after major abdominal surgery complicated by AKI.
Can Preoperative Patients Who Have a High Risk of Postoperative AKI Be Detected?
AKI affects 1–30% of patients after surgery. This case scenario raises the question of the preoperative evaluation of the risk of postoperative AKI. Several risks factors have been associated with postoperative AKI.
Cardiac Surgery Patients
Cardiovascular surgery is by far the highest risk procedure associated with postoperative AKI, with up to 30% of patients experiencing AKI. In comparison, the prevalence of AKI after major noncardiac surgery procedures 1 such as in the presented case, is approximately 1%. The preoperative estimation of the risk of postoperative AKI by an anesthesiologist relies on checking the risk factors of AKI. Most of the risk factors are nonmodifiable because they are procedure-related (urgent surgery, need for surgical reexploration, and cardiopulmonary bypass duration) or patient-related (age >70 yr, diabetes, atrial fibrillation, left ventricular dysfunction, preoperative intraaortic balloon pump, or chronic renal insufficiency). Preoperative evaluation ( e.g. , with echocardiography) of left and right ventricular functions is recommended in patients with a dyspnea of unknown origin or worsening dyspnea with a known cardiomyopathy. 3 In addition, Karkouti et al. 4 identified a per-cardiopulmonary bypass hematocrit of less than 20% and erythrocyte transfusion as potential modifiable risk factors for postoperative AKI. These findings are in line with experimental data showing the impact of normovolemic hemodilution promoting renal hypoxia. 5 However, perioperative erythrocyte transfusion was associated with an increased risk of AKI. The negative impact of erythrocyte transfusion supports the poor tolerance of multiple morphological and functional changes induced by erythrocyte storage (less deformability, depletion of 2, 3-diphosphoglycerate, inflammation, and decrease of bioavailability of nitric oxide with the liberation of free hemoglobin). These storage-induced modifications may induce a poor restoration of microcirculatory oxygenation associated with inflammation and changes in immune status. These observations emphasize the need for strategies that limit perioperative anemia and transfusion. 6
The presence of proteinuria in the preoperative period, which is easily detected by dipsticks, can indicate a risk of AKI. Mild (trace to 1+) or heavy (2+ to 4+) proteinuria has been associated with increased odds of the postoperative need for renal replacement therapy (odds ratio 7.29; 95% CI, 3.00–17.73) and mortality after cardiac surgery (hazard ratio: 1.88 for mild and 2.28 for heavy proteinuria). 7
Noncardiac Surgery Patients
In a large monocentric prospective study, Kheterpal et al. 1 identified age, emergent surgery, liver disease, high body mass index, high-risk surgery ( i.e. , surgeries with the potential for large fluid shifts or blood loss), peripheral vascular occlusive disease, and chronic obstructive pulmonary disease as independent preoperative risk factors for postoperative AKI after major noncardiac surgery in patients with previously normal renal function (defined as creatinine clearance >80ml/min). The authors created a predictive model of postoperative AKI with reasonable sensitivity and specificity but insufficient predictive values for a single patient-centered prediction. Finally, patients with poor preoperative physiological conditions, estimated by the classification of the American Society of Anesthesiologists as class IV or V, were found to be at high risk for AKI. 8 In our case scenario, three risk factors were present: age, hypertension, and intraperitoneal surgery with large fluid losses.
Diagnosis of AKI
AKI is defined by a decrease of glomerular filtration rate (GFR). AKI is defined under the Risk, Injury, Failure, Loss, and End-stage Kidney (RIFLE), the acute kidney injury network (AKIN), or the kidney disesase improving global outcome (KDIGO) classification 9 as an increase in serum creatinine level and decrease in urine output. The use of GFR estimation by Cockcroft–Gault or the Modification of Diet in Renal Disease formulae should be restrained to preoperative evaluation of GFR when renal function is stable because these formulae yield substantial disagreements regarding creatinine in patients with AKI. However, anesthesiologists must be aware of two important factors while interpreting serum creatinine levels. First, it takes time for serum creatinine to reach a steady state after a fall in GFR because of its large volume of distribution (~60% of total body weight). It is therefore difficult to predict the course of AKI when serum creatinine increases (in other words, when the plateau of GFR is reached). Second, fluid loading and hemodilution may underestimate the increase in serum creatinine levels. Macedo et al. 10 described a simple formula to correct serum creatinine for fluid balance and overcome this limitation (adjusted creatinine = serum creatinine × correction factor with correction factor= (hospital admission weight [kg]) × 0.6 + Σ (daily cumulative fluid balance [l])/hospital admission weight × 0.6). In the present case scenario, the correction of serum creatinine with respect to fluid overload allows reclassification as stage 1 AKI according to the AKIN classification in the immediate postoperative period, with earlier diagnoses. The baseline serum creatinine was 69 μ m and increased postoperatively to 77 μ m . This value became 94 µ m after adjustment on cumulative fluid balance, which corresponds with stage 1 AKI.
Can Postoperative AKI Be Prevented?
Successfully preventing AKI requires the correction of factors that contribute to AKI in the perioperative period, presented in figure 1 .

Schematic representation of factors contributing to the development of acute kidney injury (AKI) in the perioperative period and the risk associated with fluid overload. RAAS = renin–angiotensin–aldosterone system.
What Is the Contribution of Hypoperfusion to Postoperative AKI?
Although profound and prolonged interruption of renal blood flow leads to oxygen debt, renal ischemia, and tubular necrosis, 11 the total interruption of renal blood flow is a rare clinical scenario. Suprarenal aortic clamping, renal transplantation, renal artery thrombosis or dissection, and prolonged cardiac arrest can cause renal ischemia with parenchymal injury, including some degree of tubular necrosis. Intraoperative hypotension has been statistically associated with AKI only in patients with preoperative multiple risk factors for AKI. 12 Renal blood flow and GFR decrease with a decrease in mean arterial pressure below the lower autoregulation threshold for renal blood flow and glomerular filtration. If targeting a mean arterial pressure above 65 mmHg is not necessary for preventing the development of AKI in ICU patients with preserved autoregulation of GFR, an increase in mean arterial pressure may be necessary in other cases with impaired glomerular filtration autoregulation, such as that observed in advanced age, atherosclerosis, chronic hypertension, or diabetes. Ischemic injuries and inflammatory states, cardiopulmonary bypass, and oxidative stress are conditions prone to affect renal blood flow autoregulation. Angiotensin-converting enzyme inhibitors and angiotensin receptor blockers impair glomerular filtration autoregulation but do not impair renal blood flow autoregulation.
The so-called “prerenal azotemia” ( i.e. , with renal structural integrity) is often associated with tubular injury and might be considered a milder form of AKI on a continuum that includes more severe forms of AKI. The rapid reversibility of AKI with low sodium excretion is not sufficient to make the diagnosis of pure prerenal azotemia because transient AKI is associated with the increase of some biomarkers of renal damage. 13 Furthermore, persistent AKI cannot be designated as “acute tubular necrosis.” Kidney biopsies in the immediate postmortem period of septic shock rarely identified tubular necrosis but often identified infiltration by inflammatory cells and cellular apoptosis with vascular microthrombosis. 14 Consequently, the term “acute tubular necrosis” as it is classically used to describe a persistent AKI in an acute state seems inappropriate when histologic analysis of kidney has not been performed.
Perioperative Hemodynamic Optimization: A Goal-directed Therapy
Fluid resuscitation is central in the prevention and treatment of AKI. Futier et al. 15 observed a higher incidence of postoperative complications ( e.g. , anastomotic leak and sepsis) and lower urine output in the restrictive group compared with the liberal group, after abdominal surgery. This postoperative complication might arise in patients with a higher incidence of hypovolemia (defined as pulse pressure variation of 13% or more) and lower ScvO2 in the restrictive group because both are independently associated with postoperative complications. Unfortunately, anesthesiologists were blinded to ScvO2, an important variable to guide fluid resuscitation, and no clear mechanistic relation can be mentioned. Fasting has furthermore been shown to blunt renal blood flow autoregulation in rats. In contrast, there is also evidence from preclinical and clinical studies indicating that excessive fluid-administration strategies can induce the development of organ failure. 16 Excessive fluid resuscitation can induce transient hemodilution by increasing renal oxygen consumption while decreasing renal oxygen transport and leads to renal hypoxia. Such a decrease of renal parenchyma oxygen bioavailability may further compromise tissue oxygenation in conditions of potential renal injury. 5 In the worst scenario, fluid loading can worsen renal injury function due to renal congestion and increased intracapsular pressure. Therefore, more than the total amount of fluid administered is tailored and based on a perioperative stroke volume optimization, which may better prevent postoperative AKI. A recent review of randomized controlled trials 17 reported that fluid resuscitation based on goal-directed therapy resulted in fewer postoperative AKIs, but any additional administered fluid was limited (median: 555ml). The decrease in AKI was greatest in the 10 studies in which fluid resuscitation was the same between the goal-directed therapy and control groups. More importantly, inotropic drug use in goal-directed therapy patients was associated with decreased AKI, whereas studies not involving inotropic drugs found no effect. The greatest protection from AKI occurred in patients with no difference in total fluid delivery or use of inotropes. These and other results suggest that goal-directed therapy aiming to increase flow with volume, inotrope, or a combination might be the protective factor. This treatment has been formalized in multifaceted protocols for decision-making processes to administer fluids, inotropes, and erythrocyte transfusion. Although goals differed among studies, targeting a cardiac index more than 2.5 lmin −1 m −2 , a central venous oxygen saturation (ScvO2) of more than 70%, and/or an oxygen delivery index of more than 600 mlmin −1 m −2 appears to be a sound approach. In the present scenario, the intraoperative monitoring of cardiac output and ScvO2 would have indicated a need for inotropic support and not pure vasopressive therapy ( i.e. , neosynephrine).
Some differences between inotropes might be observed. For example, the use of dopexamine appears to efficiently improve organ blood flow and prevent an episode of AKI, whereas the infusion of dopamine did not. 18 The consequences of using vasopressor drugs on renal blood flow and renal function remain under debate because the renal hemodynamic consequences may depend on the inflammatory context. High doses of nonphysiological norepinephrine in healthy animals decreased renal blood flow and promoted renal ischemic injury. 19 However, during vasodilatory shock, the infusion of norepinephrine could restore renal perfusion pressure and increase renal conductance and renal blood flow. 20 Deruddre et al. 21 observed a decrease in the renal resistive index, likely reflecting a decrease of renal vascular resistance in septic patients when blood mean arterial pressure increased from 65 to 75 mmHg with the use of norepinephrine. Similarly, Redfors et al. 22 found that increasing blood mean arterial pressure with norepinephrine increased renal blood flow and the GFR after cardiac surgery. In a recent study we found no association between norepinephrine infusion for septic shock treatment and incidence/severity of AKI 23 Finally, it is worth mentioning that no strategy other than hemodynamic optimization has proven to protect kidney function in patients undergoing surgery. 24
How Should Fluid Administration with Urine Output Be Guided in the Perioperative Setting?
Urine output is often used to guide fluid therapy in the perioperative setting, and oliguria is considered a marker of hypovolemia. However, a transient decrease in urine output is not necessarily associated with a decrease in the GFR but may result from a normal renal adaptation to maintain homeostasis. The risk of fluid overload may occur if oliguria reflects surgical- and anesthesia-related neurohormonal adaptation with modest hypovolemia. An increase in intraabdominal pressure during laparoscopic surgery, mechanical ventilation with positive end-expiratory pressure, and pain and surgical stress with release of an antidiuretic hormone are all factors inducing antidiuresis. 25 Even a minor surgical injury can impair renal fluid elimination after fluid loading. 26 In a randomized controlled trial, urine output and postoperative creatinine serum concentration were not affected in obese patients undergoing laparoscopic surgery, who were randomly assigned to intraoperatively receive high (10 ml·kg −1 ·h −1 ; n = 55) or low (4 ml·kg −1 ·h −1 ; n = 52) volumes of Ringer’s lactate. 27 Finally, Holte et al. 28 did not observe signs of lower plasma volume when infusing 15ml/kg compared with 40ml/kg of Ringer’s lactate over 1.5h during laparoscopic cholecystectomy.
The clinical context and risk assessment of AKI appear central in the therapeutic response to oliguria in a patient. Oliguria in a patient undergoing surgery for a bowel obstruction or hemorrhage indicates associated hypovolemia requiring fluid infusion. Fluid deficit is also easily identified in patients with preoperative diarrhea or dehydration due to diuretics. Tachycardia, low mean arterial pressure, encephalopathy, capillary refill time, mottles, and cold extremities are important clinical signs of hypoperfusion, indicating the initiation of fluid resuscitation. However, resuscitation should be rapidly guided by physiological endpoints obtained by monitoring the optimization of cardiac output and central venous saturation ( fig. 2 ) during the perioperative period if oliguria persists despite initial fluid resuscitation (500–1000ml of crystalloids).
Avoid Fluid Overload and Venous Congestion in the Postoperative Period
A role of renal venous congestion in renal injury has emerged in experimental studies. In patients with acute heart failure, increased CVP was found to be associated with the progression of AKI, whereas cardiac output did not show this association. 29 Damman et al. 30 also found that increased CVP was associated with a reduced GFR. Interestingly, the negative impact of increased CVP is additive to compromised renal blood flow due to low cardiac output. 30 In acute lung injury, a restrictive fluid-administration strategy for surgical patients (CVP ≤4 mmHg and pulmonary artery occlusion pressure ≤8 mmHg) in the absence of shock and oliguria with a cardiac index of more than 2.5 l·min −1 ·m −2 resulted in more ventilator- and ICU-free days compared with a liberal strategy, 31 but was not associated with more episodes of severe AKI.
A relationship between fluid overload and mortality in critically ill patients was recently reported. The recent post hoc analysis of the Vasopressin in Septic Shock Trial study reported that a positive fluid balance and increased CVP were associated with increased risk of death in patients with septic shock. 32 However, the survival rate improved when the fluid balance was positive in patients with a CVP of less than 8 mmHg, suggesting that only excessive fluid restriction may be deleterious. In a randomized control trial of critically ill patients, achieving supranormal values for the cardiac index or normal values for mixed venous oxygen saturation did not reduce the incidence of acute renal failure or reduce morbidity or mortality among critically ill patients. 33
These observational studies highlight the importance of CVP monitoring in patients with heart failure or hemodynamic instability, who are undergoing major surgery. Observing the response of CVP to a fluid challenge is important because it provides information on the reach of the limit of cardiac compliance, which leads to the potential halting of fluid administration to avoid the risk of venous congestion and further organ damage. 34
Which Fluid Solution Should Be Used for the Kidney?
Crystalloids..
A more physiologic chloride concentration provides the advantage of balanced solutions (Ringer’s lactate or acetate or Hartmann solution) over normal saline. Although normal saline is the solution of choice in hypochloremic states ( i.e. , vomiting, gastric drainage, and treatment with diuretics), normal saline induces hyperchloremic acidosis in patients with normal initial serum chloride concentrations in the perioperative setting. Experimental and clinical data show that increased plasma chloride concentration increases renal vascular resistance and decreases renal blood flow and a reduced GFR. This strategy to reduce chloride-containing solutions appears to prevent episodes of AKI in ICU patients. 35
Hydroxyethyl Starches Are Associated with Negative Outcome.
The fluid resuscitation of brain-dead organ donors, based on hydroxyethyl starches (HES), is associated with an increased risk of AKI in kidney transplantations. In another randomized controlled trial, Schortgen et al. 36 found that septic patients treated with HES 200/0.6 showed a higher incidence of AKI compared with patients treated with gelatins. The Efficacy of Volume Substitution and Insulin Therapy in Severe Sepsis study showed a higher incidence of AKI in septic patients treated with HES 200/0.5 compared with those treated by crystalloids. Developed HES with lower molecular weight (130 kd) have been proposed because of the expected better risk/benefit ratio. The recently published 6S and Crystalloid versus Hydroxyethyl Starch trials did not confirm these expectations because the risk of AKI persisted with smaller molecular weight HES (HES 130/0.4), which induced a higher rate of mortality and/or dialysis. 37 , 38 Together, these trial data indicate an increased risk of AKI when HES are used. Precautions can be extended to other conditions, especially with the presence of acute inflammation ( e.g. , burns, cardiopulmonary bypass, postcardiac arrest syndrome). The safety profile of HES remains matter of debate during surgery. 39 Gelatins appear to have a safer profile, but there is little evidence for the potential risk of AKI. Finally, extra physiologic plasma oncotic pressure after the administration of a large amount of hyperoncotic solutions can decrease the GFR.
Use of Biomarkers
Urine biochemistry is frequently used to diagnose prerenal azotemia and guide fluid administration in the perioperative setting and in ICU patients, suggesting that the given parameters are indicators of renal tissue integrity and preserved tubular function. Recent evidence has suggested that urine chemistry is not a reliable tool for predicting the rapid reversibility of AKI. Preserved renal tubular sodium or urea handling does not necessarily indicate an absence of renal injury. Recently, Nejat et al. 13 found that patients with suspected prerenal azotemia showed evidence of structural injury, with increased biomarkers of renal injury. However, increased sodium excretion does not indicate tubular necrosis. Inflammation mediators have been shown to induce tubular cell dysfunction with conformational changes of the tubule Na+/H+ exchanger, urea, or chloride channels, which influence urine composition independent of any structural damage. 13 , 40 Biomarkers of renal injury ( i.e. , neutrophil gelatinase-associated lipocalin, kidney injury molecule-1) are expected to be used in diagnosis of tubular damage. 41 Many uncertainties remain regarding their validity at the bedside. The most promising biomarkers for renal injury appear to be the neutrophil gelatinase-associated lipocalin and kidney injury molecule-1. As an example, mild renal structure damage can lead to the profound loss of glomerular and/or tubular function in a patient with underlying structural alteration ( e.g. , chronic hypertension, diabetes); however, the same injury will not alter the function of an intact kidney ( fig. 3 ). A combination of biomarkers of structural injury may therefore provide a more accurate picture of renal injury compared with a single-biomarker approach.
Knowledge Gap
The optimization of systemic hemodynamics is believed to increase renal perfusion. However, the true contribution of renal hypoperfusion to the development of AKI, especially in severe sepsis and septic shock, remains a matter of debate. Intrarenal microcirculatory defects, regional and systemic inflammatory cell infiltration, and apoptosis are believed to be central in the development of AKI. Although a correlation between cardiac output and renal blood flow has been described in patients with AKI, the relationship among cardiac output, renal blood flow, renal injury, and renal function remains poorly explored. Although the development of new biomarkers of renal injury may allow the assessment of renal structure damage, tools to reliably assess renal perfusion and rapid changes in renal perfusion in patients at the bedside remain lacking. Renal Doppler of renal interlobar arteries can provide information on renal vascular resistance; however, this method does not measure renal blood flow per se . Therefore, developing tools to measure renal perfusion ( i.e. , renal blood flow and distribution of renal microvascular blood flow within the renal parenchyma) will allow a better understanding of the role of renal perfusion in renal damage.
The development of biomarkers of renal injury has been a major step forward. However, further investigation is needed to explore the significance of increased serum and urine levels of biomarkers, including neutrophil gelatinase-associated lipocalin, kidney injury molecule-1, fatty acid-binding protein 1, and α-1 microglobulin, the influence of underlying processes ( e.g. , systemic inflammation), the influence of comorbidities and their source of production ( i.e. , extra renal production), and the specificity and sensibility of assays ( fig. 4 ). A combination of methods to assess renal structural injury, renal perfusion, and renal function will likely help develop new strategies and treatments that prevent or limit the development of AKI in surgical and critically ill patients.

Proposed strategy algorithm for treatment of oliguria in the postoperative period. Left and right ventricular function should be assessed, with estimation of cardiac output and signs of congestion. Functional hemodynamic monitoring provides guidance for resuscitation with vasopressors, inotropes, fluid or blood transfusion. When no sign of ineffective circulation ( e.g. , cardiac index >2.5 l·min −1 ·m −2 , central venous oxygen saturation–ScvO 2 >75%, no clinical sign of hypoperfusion, and others) and/or presence of acute lung injury can be identified, a restrictive fluid strategy should be preferred. Depletion should be considered in case of renal congestion including high central venous pressure (CVP) ± right heart failure, tricuspid regurgitation, and dilated inferior vena cava. Finally, titration of norepinephrine based on interlobar arteries on renal Doppler has been proposed for a tailored adjustment of renal perfusion pressure. AKI = acute kidney injury; LV = left ventricle; RV = right ventricle; NSAID = nonsteroidal antiinflammatory drug; CO = cardiac output; PAC = pulmonary artery catheter.

Graphic representation of the respective contribution of chronic renal damage (comorbidities) and acute injury in the development of acute kidney injury (AKI) and the fall of glomerular filtration rate (GFR). An acute insult will lead to profound renal injury and definitive loss of function in the kidney with chronic renal damage ( e.g. , diabetes, hypertension, mild chronic renal dysfunction) while only transiently and mildly decreasing GFR in healthy kidney. Urine and/or serum level of renal injury biomarkers may help to assess the degree of structural injury to the kidney. Note that renal function may not fully recover.

Contributing factors of acute kidney injury (AKI). Note that the respective contribution of each may vary. For instance, an episode of septic AKI is mainly related to the systemic and regional inflammatory response to infection causing microvascular disorders, apoptosis, necrosis. However, a superimposed nephrotoxic agent or severe hypoperfusion can lead to further damage and/or impaired recovery. Future research should help in the understanding of the relative contribution of each factor in the development of AKI, and provide clinicians with tools to better assess the preoperative risk and help predicting the development of AKI. ACE = angiotensin enzyme converting inhibitor; CO = cardiac output; CPB = cardiopulmonary bypass; HLA = human leukocyte antigens; NADPH = nicotinamide adenine dinucleotide phosphate-oxidase.
Citing articles via
Most viewed, email alerts, related articles, social media, affiliations.
- ASA Practice Parameters
- Online First
- Author Resource Center
- About the Journal
- Editorial Board
- Rights & Permissions
- Online ISSN 1528-1175
- Print ISSN 0003-3022
- Anesthesiology
- ASA Monitor

- Terms & Conditions Privacy Policy
- Manage Cookie Preferences
- © Copyright 2024 American Society of Anesthesiologists
This Feature Is Available To Subscribers Only
Sign In or Create an Account
Thank you for visiting nature.com. You are using a browser version with limited support for CSS. To obtain the best experience, we recommend you use a more up to date browser (or turn off compatibility mode in Internet Explorer). In the meantime, to ensure continued support, we are displaying the site without styles and JavaScript.
- View all journals
- Explore content
- About the journal
- Publish with us
- Sign up for alerts
- Consensus Statement
- Published: 23 February 2023
Sepsis-associated acute kidney injury: consensus report of the 28th Acute Disease Quality Initiative workgroup
- Alexander Zarbock 1 , 2 na1 ,
- Mitra K. Nadim 3 na1 ,
- Peter Pickkers 4 ,
- Hernando Gomez ORCID: orcid.org/0000-0001-5605-3513 5 ,
- Samira Bell 6 ,
- Michael Joannidis ORCID: orcid.org/0000-0002-6996-0881 7 ,
- Kianoush Kashani ORCID: orcid.org/0000-0003-2184-3683 8 ,
- Jay L. Koyner ORCID: orcid.org/0000-0001-6873-8712 9 ,
- Neesh Pannu 10 ,
- Melanie Meersch 1 ,
- Thiago Reis ORCID: orcid.org/0000-0002-7071-117X 11 , 12 ,
- Thomas Rimmelé 13 ,
- Sean M. Bagshaw 14 ,
- Rinaldo Bellomo ORCID: orcid.org/0000-0002-1650-8939 15 , 16 , 17 , 18 ,
- Vicenzo Cantaluppi 19 ,
- Akash Deep 20 ,
- Silvia De Rosa 21 , 22 ,
- Xose Perez-Fernandez ORCID: orcid.org/0000-0002-5903-6927 23 ,
- Faeq Husain-Syed 24 ,
- Sandra L. Kane-Gill ORCID: orcid.org/0000-0001-7523-4846 25 ,
- Yvelynne Kelly 26 , 27 ,
- Ravindra L. Mehta ORCID: orcid.org/0000-0002-0908-2968 28 ,
- Patrick T. Murray ORCID: orcid.org/0000-0001-8516-1839 29 ,
- Marlies Ostermann ORCID: orcid.org/0000-0001-9500-9080 30 ,
- John Prowle ORCID: orcid.org/0000-0002-5002-2721 31 ,
- Zaccaria Ricci 32 , 33 ,
- Emily J. See 15 , 18 , 34 ,
- Antoine Schneider 35 ,
- Danielle E. Soranno 36 ,
- Ashita Tolwani 37 ,
- Gianluca Villa 38 ,
- Claudio Ronco ORCID: orcid.org/0000-0002-6697-4065 39 , 40 , 41 &
- Lui G. Forni ORCID: orcid.org/0000-0002-0617-5309 42 , 43
Nature Reviews Nephrology volume 19 , pages 401–417 ( 2023 ) Cite this article
52k Accesses
78 Citations
529 Altmetric
Metrics details
Acute kidney injury
- Prognostic markers
Sepsis-associated acute kidney injury (SA-AKI) is common in critically ill patients and is strongly associated with adverse outcomes, including an increased risk of chronic kidney disease, cardiovascular events and death. The pathophysiology of SA-AKI remains elusive, although microcirculatory dysfunction, cellular metabolic reprogramming and dysregulated inflammatory responses have been implicated in preclinical studies. SA-AKI is best defined as the occurrence of AKI within 7 days of sepsis onset (diagnosed according to Kidney Disease Improving Global Outcome criteria and Sepsis 3 criteria, respectively). Improving outcomes in SA-AKI is challenging, as patients can present with either clinical or subclinical AKI. Early identification of patients at risk of AKI, or at risk of progressing to severe and/or persistent AKI, is crucial to the timely initiation of adequate supportive measures, including limiting further insults to the kidney. Accordingly, the discovery of biomarkers associated with AKI that can aid in early diagnosis is an area of intensive investigation. Additionally, high-quality evidence on best-practice care of patients with AKI, sepsis and SA-AKI has continued to accrue. Although specific therapeutic options are limited, several clinical trials have evaluated the use of care bundles and extracorporeal techniques as potential therapeutic approaches. Here we provide graded recommendations for managing SA-AKI and highlight priorities for future research.
Similar content being viewed by others
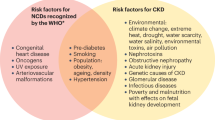
Chronic kidney disease and the global public health agenda: an international consensus
Anna Francis, Meera N. Harhay, … International Society of Nephrology
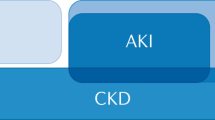
John A. Kellum, Paola Romagnani, … Hans-Joachim Anders
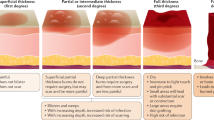
Burn injury
Marc G. Jeschke, Margriet E. van Baar, … Sarvesh Logsetty
Introduction
Sepsis is characterized by a dysregulated host response to infection that leads to life-threatening organ dysfunction, commonly including acute kidney injury (AKI) 1 . Sepsis accounts for 45–70% of all cases of AKI among critically ill patients 2 , 3 . Sepsis-associated AKI (SA-AKI) portends a worse prognosis than either syndrome in isolation 3 , 4 and is associated with longer intensive care unit (ICU) and hospital stays, higher mortality, increased rate of long-term disability and reduced quality of life in adult and paediatric populations 5 , 6 , 7 , 8 , 9 . AKI associated with sepsis can present with different phenotypes and prognoses 10 , 11 . Many aspects of SA-AKI remain poorly described, especially in the paediatric population, including its clinical definition, epidemiology, pathophysiology, impact of resuscitative and fluid strategies, role of biomarkers in risk stratification, diagnosis, and treatment guidance, and the effect of extracorporeal and novel therapies on patient outcomes. The 28th Acute Disease and Quality Initiative ( ADQI ) was aimed at identifying these knowledge gaps in both the adult and the paediatric populations, propose definitions, develop a common framework for further research in this important area, and provide recommendations for clinical practice.
The Conference Chairs of the 28th ADQI consensus committee (L.G.F., A.Z., M.K.N. and C.R.) convened a diverse panel of adult and paediatric clinicians and researchers representing relevant disciplines — critical-care medicine, anaesthesiology, nephrology and pharmacology — from Europe, North and South America, and Australia, to discuss SA-AKI. The conference was held over 2.5 days in Vicenza, Italy, on 17–19 June 2022. This consensus meeting followed the established ADQI process and used a modified Delphi method to achieve consensus, as previously described 12 , 13 . Briefly, the ADQI approach uses methods that involve a combination of both expert panel and evidence appraisal, and this approach was chosen to achieve the best of both options. Each ADQI conference is divided into three phases: pre-conference, conference, and post-conference. In the pre-conference phase, the groups that are assigned to specific topics identify a list of key questions, conduct a systematic literature search, and generate a bibliography of key studies. Studies are identified via Medline search and bibliographies of review articles; searches are generally limited to articles written in English. The conference itself is divided into breakout sessions, where workgroups address the issues in their assigned topic area, and plenary sessions, where their findings are presented, debated and refined. This approach has led to important practice guidelines with wide acceptance and adoption into clinical practice. If further research is needed, the ADQI group proposes research questions that should be addressed in the future to facilitate advances in the field. Conference participants were divided into five working groups to discuss the epidemiology and definition of SA-AKI; the pathophysiology of SA-AKI and novel underlying mechanisms; the use of fluids and resuscitative strategies to treat SA-AKI; the use of biomarkers for aiding diagnosis and guiding therapy, and in the design of clinical trials; and the use of extracorporeal treatments and novel therapies. Members of the five workgroups reviewed the literature systematically and, where possible, developed a consensus that was backed by evidence, and proposed a research agenda to address important unanswered questions. In addition, the members were asked to note the level of evidence for all consensus statements using the Grades of Recommendation Assessment, Development and Evaluation system 14 . In several cycles of presentations, feedback and adjustments, all of the individual workgroups presented their output to conference participants. The final output was then assessed and aggregated in a session attended by all attendees, who formally voted and approved the consensus recommendations.
Definition and epidemiology of SA-AKI
Definition of sa-aki and sepsis-induced aki.
Currently, no universally accepted definition of SA-AKI exists 15 . To support clinical guidelines, quality improvement initiatives, and future research, we propose that the presence of both sepsis (as currently defined in adults by the Sepsis-3 criteria) and AKI (as presently defined by the Kidney Disease: Improving Global Outcomes (KDIGO) criteria) should define SA-AKI 1 , 16 (Box 1 ). SA-AKI is a heterogeneous syndrome that occurs as the consequence of either direct mechanisms related to infection or the host response to infection, or indirect mechanisms driven by unwanted sequelae of sepsis or sepsis therapies 17 . As such, the term SA-AKI operationally unifies the presence of AKI (according to clinical, biochemical and functional criteria) in the context of sepsis as a specific disease phenotype that is characterized by a specific trajectory and outcome 18 , 19 .
Sepsis-induced AKI (SI-AKI) can be considered to be a subphenotype of SA-AKI, in which sepsis-induced mechanisms drive kidney damage directly. Thus, by definition, SI-AKI excludes injury that primarily develops as the indirect consequence of sepsis or sepsis therapies (for example, AKI caused by antimicrobial agent-induced nephrotoxicity or abdominal compartment syndrome) 20 , 21 . Importantly, mechanisms that underlie cellular and organ injury in ischaemic AKI or nephrotoxic AKI, such as microcirculation failure, inflammation and mitochondrial injury, might also contribute to SI-AKI. The limited availability of clinical tools such as biomarkers that can aid early identification complicate the distinction between SI-AKI and other causes of SA-AKI. Of note, although the development of AKI is associated with an increased risk of infection, this definition intentionally excludes sepsis following an AKI event, as the aetiology is likely different from that of SA-AKI.
To capture the temporal relationship between the two conditions, SA-AKI should be considered when AKI occurs within 7 days of sepsis diagnosis, and can be further differentiated into early (AKI occurs up to 48 h after sepsis diagnosis) or late SA-AKI (AKI occurs between 48 h and 7 days of sepsis diagnosis), to align with current AKI criteria (Box 1 ). The rationale for the proposed 7-day window in the definition of SA-AKI is based on the observation that, in most cases of sepsis, AKI occurs within a few days of sepsis onset and consensus was that AKI occurring after this timeframe was probably not directly related to the initial septic insult. The rationale for establishing a separation between early and late presentation is based on the observation that the development of AKI late in the course of sepsis is associated with worse clinical outcomes and increased mortality compared with early AKI development 22 . Distinguishing early versus late SA-AKI might improve phenotyping for targeted assessments and management, as patients with sepsis that is untreated or early in the course of treatment are more likely to have SI-AKI, whereas in patients who have received sepsis-related interventions, other factors might have also contributed to AKI development.
Box 1 Definition and epidemiology of SA-AKI
Consensus statement 1a
We propose that sepsis-associated acute kidney injury (SA-AKI) be characterized by the presence of both consensus sepsis criteria (as defined by Sepsis-3 recommendations) and AKI criteria (as defined by Kidney Disease: Improving Global Outcomes recommendations) when AKI occurs within 7 days from diagnosis of sepsis (not graded).
Consensus statement 1b
We suggest that sepsis-induced AKI should be considered a subphenotype of SA-AKI in which sepsis is the predominant driver of tissue damage (not graded).
Consensus statement 1c
We suggest that AKI diagnosed within 48 h of the diagnosis of sepsis be defined as early SA-AKI, whereas AKI occurring between 48 h and 7 days of sepsis diagnosis be classified as late SA-AKI (not graded).
Consensus statement 1d
The epidemiology of SA-AKI varies and depends on the patient population and the criteria used to define AKI and sepsis (not graded).
Epidemiology of SA-AKI
Sepsis and AKI are common in the setting of critical illness, with 25–75% of all AKI being associated with sepsis or septic shock globally 23 , 24 , 25 , 26 , 27 . The epidemiology of SA-AKI is highly variable owing to the lack of a standardized definition for SA-AKI, the loose implementation of standardized nomenclature for sepsis and AKI, the diversity of clinical settings and patient populations, and the inconsistent reporting of relevant outcomes (Supplementary Table 1 ). A 2020 systematic review of observational studies in SA-AKI illustrates these challenges in describing SA-AKI epidemiology 15 . Of the 47 studies identified, four definitions of sepsis and three definitions of AKI were used. Several studies did not report sepsis criteria, and only a few included the urine output criteria to define AKI or reported the timing of AKI relative to the onset of sepsis. Moreover, the patient populations were considerably heterogeneous, with varying incidence of sepsis, severe sepsis and/or septic shock, as well as differences in the clinical settings, which included the emergency department, medical, surgical and general ICUs, and medical wards (Box 1 ). The study also identified several risk factors for SA-AKI 15 which included the presence of septic shock, the use of vasopressors and mechanical ventilation, Gram-negative bacteraemia, use of renin–angiotensin–aldosterone system inhibitors, presence of chronic liver disease and chronic kidney disease (CKD), pre-existent hypertension and diabetes, and smoking. The reported incidence of SA-AKI ranged from 14–87% and the association with mortality (including ICU mortality, hospital mortality, 28-day and 90-day mortality) was also highly variable, ranging from 11 to 77%.
Research questions
What is the epidemiology of SA-AKI based on the proposed definition?
What is the epidemiology and the clinically relevant time frame for early versus late SA-AKI?
What are the aetiology, incidence and severity, risk factors, and renal and non-renal outcomes of both SA-AKI and SI-AKI?
How can the proposed definition of SA-AKI be operationalized in electronic health records?
Pathophysiology of SA-AKI and novel mechanisms
Mechanisms underlying the development of sa-aki.
Depending on the interaction between genotype and exposures, SA-AKI can lead to a variety of clinical phenotypes (that is, observable disease characteristics) and sub-phenotypes. Moreover, multiple pathophysiological mechanisms of injury (that is, the disease endotype) might underlie the same disease phenotype 18 , 19 (Box 2 ). This heterogeneity complicates the assessment of therapeutic efficacy in clinical trials of sepsis interventions, as different therapies might only be beneficial in the treatment of specific disease endotypes. Importantly, multiple pathophysiological mechanisms might simultaneously lead to AKI in an individual patient with sepsis. Therefore, the ability to identify the specific SA-AKI endotypes will be crucial to the development of effective therapies. Multiple mechanisms can contribute to injury in SA-AKI (Box 2 ), including systemic and renal inflammation, complement activation, RAAS dysregulation, mitochondrial dysfunction, metabolic reprogramming, microcirculatory dysfunction and macrocirculatory abnormalities (Fig. 1 ). Several additional processes might indirectly contribute to SA-AKI, such as exposure to nephrotoxic drugs, hyperchloraemia and abdominal compartment syndrome. Of note, some of these mechanisms might have temporal association with the onset and treatment of sepsis. The ability to recognize and link endotypes, subphenotypes and phenotypes therefore represents a major future research focus 10 , 28 , 29 . The biological and clinical characterization of endotypes and of the interactions between endotypes and sepsis-related treatments will be the key to refining the definitions of SI-AKI and SA-AKI set forth in this manuscript.
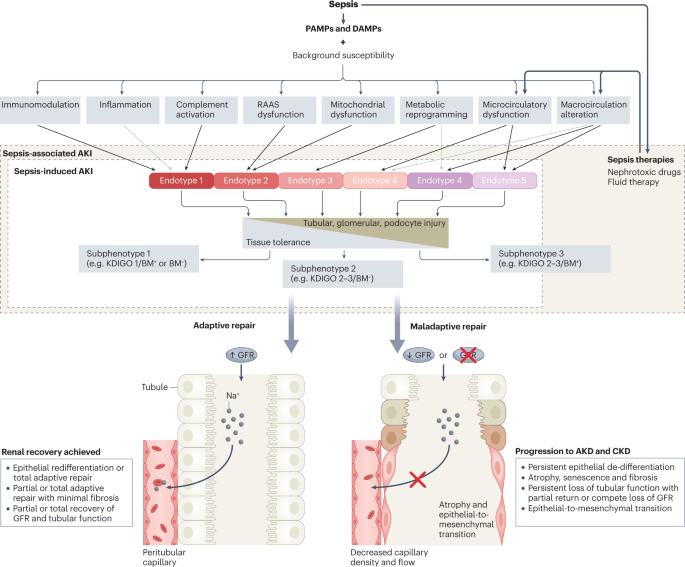
The release of pathogen-associated molecular patterns (PAMPs), such as lipopolysaccharide, and of damage-associated molecular patterns (DAMPs) from injured cells and tissues can lead to the dysregulated activation of the immune system that characterizes sepsis. Background susceptibility to tissue and organ injury varies across individuals, according to non-modifiable factors such as comorbidities, current lifestyle choices (for example, smoking), genetic variants (for example, single nucleotide polymorphisms), premorbid comorbidities and medication use (for example, the use of renin–angiotensin–aldosterone system (RAAS) inhibitors for blood pressure control), and modifiable factors such as the use of vasopressors, mechanical ventilation or the presence of bacteraemia. The pathways induced in response to sepsis (or sepsis therapies) are modulated by background susceptibility and determine the endotype-defining pathophysiological mechanisms that underlie acute kidney injury (AKI) in patients with sepsis. The combination of different disease mechanisms can therefore result in a variety of disease endotypes. Sepsis-associated AKI (SA-AKI) includes cases of sepsis-induced AKI, whereby the response to sepsis causes kidney injury directly, as well as cases in which other sepsis-associated factors (such as therapeutic interventions) indirectly contribute to AKI. For example, very high doses of norepinephrine can decrease microvascular blood flow and exacerbate the microvascular dysfunction induced by sepsis. Several tissue tolerance mechanisms, such as the activation of haem-oxygenase 1 or mitochondrial autophagy (that is, mitophagy), can protect cells and tissues from injury caused by PAMPs and DAMPs. Importantly, the disease phenotypes observed in the clinic (for example, sub-phenotypes 1–3) reflect a complex interplay between background susceptibility, disease endotypes and tolerance capacity. Consequently, phenotypes cannot be directly traced to a specific disease mechanism or endotype, and therefore clinical subphenotyping of patients with sepsis might not be sufficient to identify relevant therapeutic targets. The figure is a simplified representation of these complex interactions but also illustrates a roadmap for investigating mechanism-specific biomarkers that can identify whether specific endotypes and tolerance mechanisms are operational, thereby enabling the development and assessment of mechanism-specific therapies. BM, biomarker; AKD, acute kidney disease; CKD, chronic kidney disease; GFR, glomerular filtration rate; KDIGO, Kidney Disease Improving Global Outcomes.
Box 2 Pathophysiology of SA-AKI
Consensus statement 2a
Sepsis-associated acute kidney injury (SA-AKI) is a heterogeneous syndrome as multiple mechanisms contribute to injury with varying intensity between and within patients across the course of sepsis (not graded).
Consensus statement 2b
The relative contribution of one or more specific mechanisms that lead to injury defines distinct sepsis-induced AKI endotypes (not graded).
Consensus statement 2c
Modifiable and non-modifiable factors confer susceptibility to SA-AKI and determine the severity of AKI as well as the trajectory of recovery (not graded).
Consensus statement 2d
Integrating mechanism-specific biomarkers with clinical information will enable the identification of specific endotypes of SA-AKI (not graded).
Consensus statement 2e
Identifying distinct endotypes of SA-AKI might provide crucial prognostic information, help to define treatment responsiveness and enrich clinical trial populations (not graded).
Determinants of susceptibility and recovery trajectory
Several modifiable and non-modifiable factors affect susceptibility to AKI and disease severity in patients with sepsis. As discussed earlier, a 2020 meta-analysis identified ten clinical risk factors with prognostic value 15 . Although useful for risk stratification, such clinical factors only partly explain an individual’s susceptibility to developing SA-AKI and do not consider susceptibility within the conceptual framework of different SA-AKI endotypes.
Genetic and epigenetic variability, as well as the interplay between resistance and tolerance mechanisms during sepsis, have been recognized as potential key factors underlying individual susceptibility (Box 2 ). In patients with sepsis, single nucleotide polymorphisms (SNPs) in genes involved in both inflammatory ( TNF , IL6 and IL10 ) 30 , 31 , 32 , 33 and vascular ( VEGF ) 34 pathways have been implicated in the development of AKI 35 , 36 . However, study results have been inconsistent and three independent systematic reviews did not find a clear link between specific genetic variants and AKI risk in patients with sepsis 37 , 38 , 39 . Epigenetic control of gene expression is mediated by enzymatic DNA methylation or histone modification without changes in the genetic code. This type of control has been implicated in the induction of cross-tolerance in immune cells and kidney tubular epithelial cells, whereby innate and adaptive immune responses to a subsequent insult are attenuated 40 , 41 , 42 , 43 . However, exposure to sublethal ischaemic or toxic AKI can also be followed by a local hyper-inflammatory response in animals subsequently challenged with lipopolysaccharide or lipoteichoic acid 44 , 45 . This ‘biological memory’ and the capacity to reprogram future responses is probably induced by epigenetic mechanisms, whereby histone-modifying enzymes enhance the expression rate of inflammatory genes 45 , 46 , 47 . The influence of a previous insult on the response to a second insult are likely dependent on both the extent of the initial insult and the timing in relation to the initial event. Resistance and tolerance capacity (not to be confused with cross-tolerance described above) might explain an individual’s susceptibility to SA-AKI. Resistance capacity refers to the ability of the immune system to control or eliminate the microbial burden, whereas tolerance capacity has a critical role in sepsis because it reflects the ability of a cell, tissue, or organ to attenuate its susceptibility to injury during infection 48 , 49 . Tolerance mechanisms protect the host from the potential harm associated with resistance mechanisms. Several protective tolerance mechanisms against AKI have been identified including in preclinical models of malaria 50 , 51 , viral and bacterial sepsis 52 , 53 , 54 , ischaemia–reperfusion injury, and nephrotoxicity 50 , 51 , 52 , 53 , 54 . Tolerance mechanisms seem to be specific to the type of insult or infection and are thus not entirely generalizable. For instance, starvation protects from tissue injury and death in rodents with bacterial sepsis but worsens outcomes in viral sepsis 55 .
Similar to individual susceptibility to AKI, the trajectory of post-AKI recovery — determined by adaptive or maladaptive repair processes — is influenced not only by genetic variation, but also injury severity, recurrent insults, and the presence of underlying CKD. Within the nephron, adaptive repair involves the proliferation and re-differentiation of tubular epithelial cells, as well as the repair and regeneration of endothelial cells. By contrast, maladaptive repair manifests as tubular atrophy and dilation 56 , expansion of interstitial fibroblasts and myofibroblasts 57 , endothelial-to-mesenchymal transition and a reduction in peritubular capillary density 58 , 59 . Together, these maladaptive processes culminate in interstitial fibrosis, tissue hypoxia, increased oxidative stress and accelerated senescence 56 . Progressive fibrosis is followed by loss of functional renal reserve, glomerular hypertension and the development of CKD 60 .
SA-AKI mechanisms and novel therapeutic targets
As mentioned above, many clinical studies have attempted to investigate the benefit of therapeutic interventions in unselected patient populations, which might have reduced therapeutic efficacy signals and led to negative results. The framework proposed in this consensus statement advocates for a strategic shift in randomized controlled trial (RCT) design, whereby the deployment of any therapeutic strategy is targeted to subgroups of patients defined according to disease likelihood endotypes or therapy-responsive subphenotypes to enhance the possibility of discovering effective therapies. In addition, endotyping and subphenotyping will provide a platform to better understand the interaction between pathogenic mechanisms induced by sepsis directly or by sepsis-related factors (for example, nephrotoxins or complications such as abdominal compartment syndrome). Moreover, this granular approach will help to define the relationship between pathogenic mechanisms and time, and the therapeutic potential of different interventions in early and late SA-AKI or SI-AKI (Box 2 ).
Several interventions that modulate pathogenic processes involved in SA-AKI have been tested. For example, anti-inflammatory agents were not found to be beneficial but post hoc analyses demonstrated that dexamethasone was associated with a reduced need for kidney replacement therapy (KRT) in patients with sepsis 61 . A phase II trial showed long-term kidney benefit and lower mortality in patients who received the anti-inflammatory recombinant alkaline phosphatase ( NCT04411472 ) 62 . With regard to haemodynamics and oxygen delivery, studies using angiotensin 2 (ref. 63 ) (ASK-IT trial, NCT00711789 ) and levosimendan 64 , 65 suggest that these agents might protect the kidneys. Of note, although mitochondrial dysfunction is a feature of SA-AKI, no compounds targeting this impairment are in the clinical development phase thus far. Interventions related to cellular repair and fibrosis, including mesenchymal stem cell therapy 66 , protein-7 agonist 67 and mimetics of hepatocyte growth factor 68 , have been studied but not yet found to decrease the incidence or severity of AKI.
How can we validate mechanisms recognized in preclinical models in the clinical setting?
How can we identify distinct endotypes of SA-AKI?
How can we leverage molecular diagnostic technologies to identify novel therapeutic targets?
How can we match distinct endotypes of SI-AKI to targeted therapies?
How can we optimize the delivery of novel therapies to maximize efficacy within the kidney while minimizing remote toxicity?
What is the role of damage and systemic markers of sepsis in defining the mechanism and time course of SA-AKI and its endotypes?
Fluid and resuscitation therapy
Goals of fluid management in sa-aki.
Restoring intravascular volume through redistribution of fluid is a therapeutic target in sepsis to sustain adequate perfusion and tissue oxygen delivery. Together with source control and treatment with antimicrobials, the administration of fluids and vasopressors are key management strategies in SA-AKI. The main goal of fluid administration is to increase preload and cardiac output to maintain adequate oxygen delivery to vital organs. The haemodynamic targets for SA-AKI should be consistent with those outlined in the Surviving Sepsis Campaign Guidelines 2021 and our previous report on haemodynamics for monitoring fluid therapy from the 12th ADQI Consensus Conference 69 , 70 (Box 3 ). The utility of central venous pressure (CVP) as a haemodynamic indicator in SA-AKI is unclear and, although a high CVP might reflect congestion in the capacitance vessels 71 , 72 , CVP correlates only moderately with overall volume status, given that CVP is also influenced by right ventricular function 73 . Assessment of fluid status and response to fluid administration (that is, fluid responsiveness) should be undertaken to prevent under- or over-hydration. Urine output should be closely monitored but should not be used to guide fluid therapy in patients with SA-AKI. Measurement of intra-abdominal pressure can be useful in patients at risk of AKI. Daily and cumulative fluid balance should inform fluid management in patients with SA-AKI, as many studies have shown that fluid overload in critically ill patients is associated with excess mortality 74 , 75 . Assessment of fluid responsiveness should include clinical perfusion markers, and advanced haemodynamic monitoring, invasive or non-invasive, where available, should be considered 76 . Of note, the rate and duration of intravascular volume expansion following fluid administration are crucial given the role of the endothelial glycocalyx layer in vascular permeability where injury to this layer might lead to increased rates of fluid loss from the intravascular into the extravascular space and further fluid administration could cause fluid overload 77 , 78 , 79 .
Box 3 Fluid management in SA-AKI
Consensus statement 3a
In patients with sepsis-associated acute kidney injury (SA-AKI), haemodynamic management should be similar to that recommended by the Surviving Sepsis Guidelines 69 (grade 2C).
Consensus statement 3b
The significance of central venous pressure as a marker of congestion in SA-AKI is uncertain, although a high central venous pressure has been associated with AKI. Therefore, we suggest using measures of fluid status assessment and fluid responsiveness to assess the need for fluid administration (grade 1C).
Consensus statement 3c
We recommend daily and cumulative fluid balance monitoring (grade 1C) with concurrent, non-kidney organ dysfunction to inform fluid management strategy in SA-AKI (grade 2C).
Consensus statement 3d
We recommend that the amount of fluid administered in SA-AKI be targeted to specific endpoints (grade 1B).
Consensus statement 3e
We recommend that fluid protocols and frequency of monitoring urine output and kidney function consider the severity and rate of progression of AKI (grade 1C).
Consensus statement 3f
We recommend that the choice of fluids be informed by the need to correct patient acid–base and electrolyte imbalances (grade 1C).
Consensus statement 3g
We suggest that balanced solutions and 0.9% saline be used for resuscitation based on the biochemical profile of individual patients while their biochemical effects are closely monitored (grade 2B).
Consensus statement 3h
Albumin and bicarbonate might be of benefit in SA-AKI (grade 1C), but we recommend against the use of starch, gelatin and dextran (grade 1A).
Consensus statement 3i
We recommend the administration of vasopressors, inotropes, and diuretics based on haemodynamic assessments, phase of sepsis, and the severity of AKI (grade 1B).
Consensus statement 3j
We recommend that norepinephrine be used as the first-line vasopressor for sepsis with organ dysfunction (grade 1A).
Consensus statement 3k
We suggest that combining vasopressors with volume administration might have a net fluid-sparing effect (grade 1C).
Consensus statement 3l
We recommend the use of diuretics in patients with fluid overload (grade 1C).
Consensus statement 3m
We suggest that some subtypes of SA-AKI might benefit from the use of specific vasopressors (for example, vasopressin or angiotensin 2) (grade 2B).
Role of fluid protocols for the treatment of SA-AKI
Given the need to manage fluid volume, composition and distribution concurrently with AKI and sepsis of varying severity, the potential for toxicity from fluid therapy is substantial. The fluid management goals can be protocolized, utilizing the type, rate and duration of fluid delivery to target the interdependent relationship between sepsis and AKI (Box 3 ). Early and late SA-AKI might require different treatment protocols. Whereas haemodynamic stabilization is a priority in early SA-AKI, targeting fluid overload might be more relevant in late SA-AKI. The ongoing CLOVERS trial and ARISE FLUIDS observational study are expected to provide additional evidence in this field 80 , 81 . The use of fluid-restrictive protocols is feasible in SA-AKI, but a beneficial effect has not yet been demonstrated. The REVERSE-AKI trial, suggested that restricted daily fluid intake, aiming for a negative daily fluid balance with unrestricted use of diuretics was associated with reduced use of KRT compared with usual care. However, only ~50% of participants with AKI also had sepsis 82 . The recently published CLASSIC trial found that intravenous fluid restriction after initial fluid resuscitation was not superior to liberal fluid management with regard to kidney outcomes in adult patients with septic shock in the ICU 83 . As described previously at the 12th ADQI conference, the four phases of intravenous fluid therapy — resuscitation, optimization, stabilization and de-escalation — form an appropriate conceptual framework for tailoring fluid therapy to the individual patient context 70 . Although (balanced) crystalloids are often used, the recent BaSICS and PLUS trials and meta-analysis found no clinical benefit of balanced solutions over the use of 0.9% saline solutions 84 , 85 , 86 , 87 . The SMART trial reported that the use of balanced crystalloids significantly decreased major adverse kidney events at day 90 (MAKE 90 ) and a composite end point (death, KRT, or persistent kidney dysfunction) compared saline; however, only ~15% of the patient population had sepsis at baseline 88 . Similarly, the SALT-ED trial noted a significant decrease in MAKE 90 with the use of balanced crystalloids versus saline, but the study cohort comprised a heterogenous population of patients receiving treatment in the emergency department 89 .
Colloids of high molecular weight theoretically cause selective expansion of the intravascular space but this effect is impaired when vascular permeability is altered and the endothelial glycocalyx is damaged in inflammation. Supplemental albumin administration, as a preferred colloid over synthetic colloids, might be considered if substantial fluid replacement is required; however, to date, no data support its routine use for volume resuscitation in sepsis and data to inform a suggested cut-off value for crystalloid infusion above which albumin should be considered as part of resuscitation fluid are limited 69 , 85 , 90 , 91 . According to the subgroup analyses of patients with severe sepsis or septic shock in the SAFE and ALBIOS trials, the administration of albumin, either as a primary resuscitation fluid or as a supplement to crystalloid resuscitation, might be associated with a lower mortality trend 85 , 90 . However, these were post hoc analyses and must be interpreted with caution. We await the results of the ongoing ALBumin Italian Outcome Septic Shock-BALANCED trial (ALBIOSS-BALANCED) to provide evidence as to whether albumin, as a primary or supplemental resuscitation fluid, improves outcomes in patients with septic shock 92 . Of note, the use of hydroxyethyl starch (HES) has been associated with increased mortality risk and other adverse outcomes compared with crystalloids, including the need for KRT in patients with severe sepsis; accordingly, the FDA has mandated changes to safety labelling in HES products and its use was suspended in the European Union 93 . Hence, we recommend against the use of HES for fluid resuscitation in patients with SA-AKI. Similarly, compared with crystalloids or albumin, use of gelatin was associated with an increased risk of anaphylaxis, mortality, AKI and bleeding in a 2016 meta-analysis of 30 RCTs, 8 non-randomized studies and 22 animal studies 94 . Dextrans have also been associated with anaphylaxis, coagulation disorders, osmotic nephrosis and AKI in observational studies 95 , 96 . The BICAR-ICU study found that treatment with intravenous 4.2% sodium bicarbonate for severe metabolic acidaemia (pH <7.20) and moderate-to-severe AKI in the ICU reduced the primary composite outcome (death from any cause by day 28 and the presence of at least one organ failure at day 7) and 28-day mortality 97 . However, only ~60% of the trial population in each study arm had sepsis at the time of randomization, so the results cannot be fully extrapolated to patients with SA-AKI, particularly as these findings are specific to patients with severe acidaemia in the presence of AKI.
Combination of adjunctive therapies with fluid management
Adjunctive therapies should be used to optimize haemodynamic status and enhance fluid management, and should be adjusted based on the clinical condition of the patient (Box 3 ). Vasoactive agents are also key to haemodynamic optimization, and their use should not be limited by the presence or absence of central venous access. If clinically required, peripheral use of vasoactive agents should be initiated with careful monitoring for extravasation. Norepinephrine remains the first-line vasopressor for sepsis and SA-AKI 69 , 98 . The early use of vasopressors might have a volume-sparing effect. Conversely, diuretics have an essential role in the treatment of volume overload, potentially reducing mortality 99 . In the FFAKI, REVERSE-AKI and RADAR-2 RCTs, forced fluid removal prevented and treated fluid overload effectively in critically ill patients 82 , 100 , 101 . However, depending on the severity of AKI, significant increases in diuretic therapy dosage might be required to achieve a sufficient effect 100 , 102 . Moreover, specific phenotypes of SA-AKI might benefit from specific vasopressors. A secondary analysis of the VASST trial 29 showed improved survival compared with norepinephrine in patients with a subphenotype of SA-AKI that was characterized by a low severity of disease and low levels of angiotensin 1, angiotensin 2 and IL-8. A posthoc analysis of the ATHOS-3 trial found that patients with vasodilatory shock and AKI requiring KRT had significantly greater 28-day survival with a higher mean arterial pressure response and a higher rate of KRT discontinuation when treated with intravenous angiotensin II compared with placebo 103 . In the case of impaired cardiac function, inotropes should be considered to optimize oxygen delivery.
What is the utility of haemodynamic monitoring in SA-AKI?
What is the role of the assessment of renal perfusion using ultrasound, measurement of intra-abdominal pressure and assessment of mean perfusion pressure in managing SA-AKI?
What is the effect of 0.9% saline versus balanced crystalloids on outcomes for patients with SA-AKI, particularly with regard to hyperchloraemia and hyper- and hyponatraemia in patients with SA-AKI?
Is there an indication for using albumin for fluid resuscitation in patients with SA-AKI?
What is the clinical utility of markers of glycocalyx damage during fluid therapy and their correlation with markers of kidney dysfunction?
Does the choice of vasopressor agent affect the course of SA-AKI?
What is the role of diuretics in treating fluid overload in SA-AKI?
Biomarkers for diagnosis and guiding treatment
Measures for sa-aki prediction and diagnosis.
The ADQI 23 Consensus Conference statement 104 proposed combining damage and functional biomarkers to increase the sensitivity of AKI definitions. For example, stage 1S (‘subclinical AKI’), could be defined by biomarker-positive evidence of kidney injury that does not meet the KDIGO criteria (that is, AKI stage 1 defined by creatinine and urine output criteria are not achieved). Data from the Protocolized Care for Early Septic Shock (ProCESS) cohort reported in 2022, demonstrate that, for a given stage of KDIGO-defined AKI, higher biomarker values (stages 1B, 2B and 3B) were associated with a higher risk of 30-day mortality than stages 1A, 2A and 3A 105 . However, 30-day survival did not differ between biomarker-positive (stage 1S) and biomarker-negative cases in the absence of KDIGO AKI criteria.
Emerging data demonstrate that plasma proenkephalin (penKid) identifies patients with sepsis who are at an increased risk of developing KDIGO-defined functional AKI and MAKE; patients with stage 1S AKI (defined by plasma penKid increases) had higher 28-day mortality than those with KDIGO-defined functional AKI 106 , 107 . Similarly, plasma cystatin C has been proposed as an alternative to serum creatinine as a functional marker of glomerular filtration rate changes to identify AKI in patients with critical illnesses, including those with SA-AKI 108 . Whether these functional markers, which have shorter half-lives than serum creatinine, provide a swifter diagnosis of decreasing kidney function, can assist in appropriate drug dosing, and/or if other biological and analytical features improve the diagnosis and prognostication of AKI in sepsis, remains unclear 109 , 110 . Functional biomarkers (for example, cystatin C and penKid) and damage or stress biomarkers (for example, neutrophil gelatinase-associated lipocalin (NGAL) and (TIMP2) × (IGFBP7)) predict SA-AKI with high accuracy 104 , 106 , 111 , 112 , 113 , 114 , 115 . Additionally, several non-biochemical tools can forecast SA-AKI, including logistic or artificial intelligence-driven prediction models based on available clinical information 116 , 117 , 118 , 119 . The clinical information used for these models includes clinical and physiological data, volume assessment and other laboratory information. These forecasting models and biomarkers could be used in combination to assign patients with SA-AKI to specific phenotypes and subphenotypes 117 , 120 , 121 (Box 4 ). Several investigations have demonstrated the clinical potential for using biological, genetic and machine-learning multidimensional models for assessing AKI risk and improving outcomes 29 , 118 , 119 . Further research is needed to distinguish SA-AKI from other AKI aetiologies using validated measures, including biomarkers, and to characterize and differentiate SA-AKI endotypes or sub-phenotypes (Fig. 2 ).
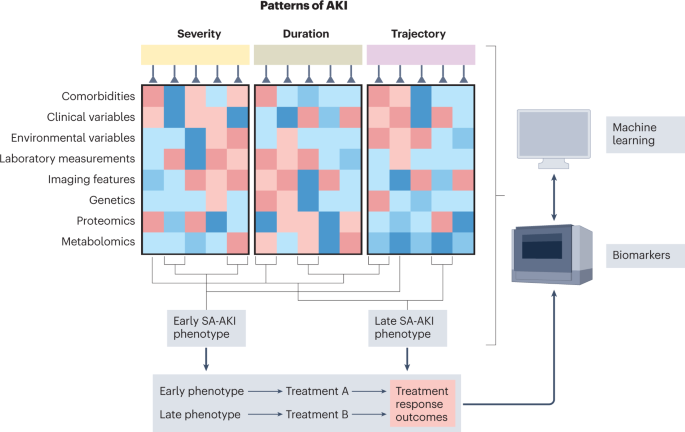
At the top are the characteristics of an individual’s acute kidney injury (AKI) journey — severity, duration and trajectory. Each episode of AKI will have unique biological characteristics. In addition to pre-sepsis comorbidities, several tests will be performed, including standard laboratory measures, advanced biomarker assessment and genetic, proteomic and metabolic tests. When combining these tests with clinical and environmental factors, distinct sepsis-associated AKI (SA-AKI) phenotypes will be characterized, each with its distinct course and response to treatment plans. In the near future, biomarkers and machine-learning algorithms might be used to characterize patients by phenotype and endotype more rapidly to optimize their care or expedite enrolment into clinical trials. Adapted from Maslove et al. 193 , Springer Nature Limited.
Box 4 Biomarkers for diagnosis and guiding treatment in SA-AKI
Consensus statement 4a
We suggest the complementary use of validated measures — including functional, stress and tissue damage-related biomarkers — be considered in combination with the consensus Kidney Disease Improving Global Outcomes (KDIGO) definition to diagnose sepsis-associated acute kidney injury (SA-AKI) (grade 2C).
Consensus statement 4b
We recommend that measures validated to predict an episode of AKI in patients with sepsis be used in combination with available clinical information (grade 1B).
Consensus statement 4c
We suggest that selected functional and stress- or injury-related biomarkers should be used for clinical assessment to identify and discriminate patients with sepsis at risk of transient or persistent SA-AKI. These biomarkers can also enhance the risk assessment of the severity, duration, trajectory of recovery and occurrence of non-renal outcomes in patients with established SA-AKI (grade 1B).
Consensus statement 4d
We suggest that sepsis biomarkers be used to complement functional and tubular injury-related biomarkers for the prognosis of early or late SA-AKI (grade 2C).
Measures for SA-AKI course and outcome prediction
The duration of AKI has gained relevance as an additional dimension in AKI phenotyping 122 , given that a pattern of persistent, non-resolving AKI is associated with poorer short-term 20 and long-term outcomes 123 , 124 , 125 than transient AKI, regardless of disease severity. Early patient risk identification could aid the development of personalized in-hospital 126 and outpatient 127 care strategies to reduce AKI progression, the development of AKI-related complications and the risk of sequelae of SA-AKI, as well as enabling predictive enrichment in randomized trials of potential therapeutic targets. Various markers, including clinical risk scores, functional, stress- and injury-related biomarkers, and imaging tests, have been described in patients admitted to the ICU (Tables 1 and 2 ). Notably, many markers were tested in heterogeneous cohorts of critically ill patients; thus, their generalizability to patients with sepsis might require further investigation. Scoring systems (for example, the renal angina index) and imaging tests, such as the renal resistive index, can be considered complementary to direct AKI biomarker testing to optimize their use for the prediction of persistent AKI and other outcomes 128 , 129 (Box 4 ).
Various sepsis-associated biomarkers have been evaluated to assess the prognosis of SA-AKI (Supplementary Table 2 ). However, the timing of SA-AKI diagnosis relative to the timing of biomarker assessment is often highly variable, thus complicating the differentiation of early versus late SA-AKI and indeed, most sepsis-associated biomarker monitoring is anchored at the time of admission to the ICU. Of note, prognosis determination could be influenced by the introduction of a confounding variable after SA-AKI diagnosis but before the outcome measure. Many studies evaluating the association between biomarkers and AKI considered single or multiple biomarkers (Supplementary Table 3 ), but few directly compared or measured the additive impact of combining sepsis and kidney biomarkers to determine prognosis. Of note, AKI biomarkers predicting acute kidney disease (that is, 7 to 90 days post-insult) in patients with sepsis are less well-defined 130 .
Do kidney injury biomarkers add prognostic discrimination in patients with SA-AKI, and can they identify a high-risk 1S subgroup in the absence of KDIGO-defined functional AKI (subclinical AKI)?
What is the impact of individual biomarkers on SA-AKI clinical trajectories, including severity, duration, recovery and non-kidney-related outcomes?
What are the best methods for integrating clinical information, identification of phenotypes and single or serial use of validated measures to predict clinical course and the likelihood of response to interventions?
What is the role of the measurement of sepsis and kidney markers for targeted intervention in different subphenotypes and endotypes of SA-AKI?
Extracorporeal therapies for SA-AKI
Extracorporeal blood purification in sa-aki.
Extracorporeal blood purification (EBP) can be performed using various techniques (Supplementary Fig. 1 ); the most common techniques involve systems that are mainly employed for KRT with the aim of re-establishing homeostasis (Table 3 ). These techniques affect the molecular and electrolyte composition of blood directly, which might enable the correction, replacement and maintenance of homeostasis in multi-organ dysfunction through the control of acid–base, electrolyte and fluid balances. EBP techniques might also facilitate the control of immune dysregulation in sepsis by removing endotoxins, cytokines, pathogens and inflammatory factors 131 , 132 , 133 , 134 , 135 . The selection of a specific EBP modality or combination of selected modalities should be based on the patient’s needs (Table 3 ). Global practice is very heterogeneous owing to the lack of consensus guidelines and high-grade evidence, and the limited availability and approval of specific devices and therapies.
Accepted indications for commencing KRT to support kidney function during SA-AKI are consistent with those in place for other causes of AKI 16 . Although early KRT initiation for SA-AKI has been used for fluid and solute control and to prevent multi-organ dysfunction, no clear benefit has been demonstrated for earlier initiation 136 . Of the latest RCTs focused on the timing of initiation of KRT in critically ill AKI patients 137 , 138 , 139 , the IDEAL-ICU study 140 is the only one that focused on SA-AKI and demonstrated that earlier initiation of KRT had no significant survival benefit compared with ‘standard’ initiation, although a significant number of patients in the ‘delayed’ group were not treated with KRT, owing to spontaneous kidney recovery. Of note, a further study on the initiation of KRT in SA-AKI is currently underway 141 . Initiation of KRT in both septic and non-septic conditions should be based on clinical assessment and goals of EBP for kidney support, not just on creatinine levels and oliguria 69 . In patients with SA-AKI for whom KRT is indicated and with explicit clinical (for example, shock) and/or biological (for example, the detection of damage-associated molecular patterns and pathogen-associated molecular patterns) criteria are recognized, EBP for immunomodulatory support might be considered in combination with KRT, either concurrently (for example, hybrid treatments) or following KRT (Box 5 ). EBP for immunomodulatory support can be considered in patients with sepsis as a stand-alone treatment if kidney support is not required 142 . Despite the biological rationale for using EBP approaches in SA-AKI, namely their potential to limit the pathophysiology of organ damage, mitigate homeostatic derangements and prevent multi-organ dysfunction in sepsis, the lack of robust data precludes definitive recommendations with regard to its use in patients with sepsis or SA-AKI, including its timing in the clinical course of the disease.
Box 5 Extracorporeal and novel therapies for SA-AKI
Consensus statement 5a
Extracorporeal blood purification (EBP) techniques can be used to remove pathogens, microbial toxins, inflammatory mediators and toxic metabolites from the blood as well as replenish solutes (grade 1A).
Consensus statement 5b
Kidney replacement therapy provides organ support through solute control, blood detoxification, and fluid balance via diffusion, convection and adsorption. Peritoneal dialysis can be used for kidney support when extracorporeal techniques are unavailable (grade 1A).
Consensus statement 5c
Emergent indications for initiating kidney replacement therapy do not differ between SA-AKI and other types of acute kidney injury (grade 1A).
Consensus statement 5d
Initiation of EBP in sepsis might be considered for immunomodulatory support in patients who meet explicit and timely clinical and/or biological criteria, such as high concentrations of damage-associated molecular patterns and pathogen-associated molecular patterns, as well as other targets of systemic inflammation (not graded).
Consensus statement 5e
Optimal delivery of extracorporeal therapies is determined by factors such as timely and safe initiation, treatment duration, appropriate vascular access placement and maintenance, individualized patient dose, safe and effective anticoagulation protocols, appropriate adjustments of medications (for example, antimicrobials or vasopressors) and nutrients, and a dynamic prescription of fluid removal (not graded).
Consensus statement 5f
Safe and effective therapy requires objective indicators of treatment response, which must be evaluated throughout the therapy course with a focus on patient-centred care goals (grade 1B).
Delivery and monitoring of extracorporeal blood purification therapies
For haemoadsorption therapies, anticoagulation is recommended, and the indications for venous access are similar to those of KRT 143 . Haemoadsorption cartridges can be combined with the KRT circuit with variable blood flow rates 144 , 145 , 146 . Initiation of haemoadsorption to remove endotoxins has been based on the result of the endotoxin activity assay, which compares the activation of neutrophils caused by endotoxin to the theoretical maximum response when exogenous endotoxin is added to the blood sample 147 , 148 , 149 , 150 , 151 . Polymyxin B haemoadsorption has been used in sepsis with variable results. When Polymyxin B haemoadsorption was applied for 2-h sessions for 2 consecutive days, it was found to be safe but without a survival benefit. However, a potential survival benefit was observed in patients with an endotoxin activity assay of 0.6–0.9 EAA units, indicative of a high but measurable endotoxin burden 151 , 152 , 153 . These effects are being investigated in an ongoing trial (the TIGRIS trial, ClinicalTrials.gov Identifier: NCT03901807 ).
New synthetic polymeric resins enable highly biocompatible haemoadsorption designed for the non-specific adsorption of damage-associated molecular patterns and other mediators. The rationale for their use is based on the peak concentration hypothesis, which postulates that haemoadsorption might enable removal of the solutes with the highest concentration in blood (either pro- or anti-inflammatory mediators), helping to restore immunohomeostasis by mitigating the uncontrolled response of the innate and/or the adaptive immunity of the patient 154 , 155 . Additional research on clinical benefits is warranted. Notably, the unselective nature of such EBP interventions might result in unrecognized losses of electrolytes, nutrients and drugs. As significant losses of amino acids and several micronutrients, such as vitamins B1 and C, copper and selenium can occur, careful monitoring in prolonged KRT should be considered 156 . Importantly, discrepancies exist in the observed and predicted removal of antimicrobials with haemoadsorption in critically ill patients 157 , 158 , 159 , 160 , 161 , 162 , 163 . In patients undergoing continuous KRT, antimicrobial clearance depends on the effluent fluid rate and therapeutic drug monitoring should therefore be considered where available 157 . The identification of subphenotypes of patients and the delivery of EBP should be assessed and supported by a multidisciplinary team of trained personnel to improve patient selection and safety 164 . Optimal EBP delivery demands timely communication between stakeholders, iterative adjustment of therapy and quality assurance systems 165 , 166 . Patient selection, timing, duration and appropriate primary clinical endpoints are crucial elements for well-conducted clinical studies in this area. Moreover, given the phenotypic variability of SA-AKI, one extracorporeal therapy might be effective in a specific phenotype, while having no effect, or even causing harm, in others. Investigators should refrain from choosing mortality as the primary end point because of the well-known variation in mortality across centres, sepsis and AKI phenotypes 167 . RCTs examining the effects of EBP, in which patient heterogeneity is reduced through specific inclusion criteria with clinically relevant endpoints, including haemodynamic and organ improvement, as well as ICU stay rather than only mortality, should be performed.
How do the EBP therapies affect the pathophysiology of SA-AKI?
In which subgroup of patients, and when in the clinical course of the disease, might EBP therapies be beneficial?
Are EBP therapies safe, efficacious and cost-effective?
What meaningful target molecules can guide EBP therapy, and can their kinetics be employed to assess response to treatment?
What is the effect of EBP therapies on other organ systems during sepsis?
SA-AKI: the paediatric perspective
This Consensus statement has thus far been based on a systematic review of the literature as it pertains to adult medicine, especially with the use of Sepsis-3 as the definition of sepsis in adult patients. Although there is undoubtedly much overlap in the pathophysiology of SA-AKI throughout the lifespan, neonates and children do merit particular attention. SA-AKI is a common cause of AKI in critically ill children 168 but the definition of SA-AKI in paediatrics is currently limited by the reliance on adult sepsis criteria. The 26th ADQI recently published consensus recommendations for the advancement in paediatric AKI with particular attention to the role of development as a biological variable that modulates the development of and recovery from AKI 169 . AKI prediction in paediatric patients continues to progress. The renal angina index has been modified for paediatric patients with sepsis and shown to reliably predict SA-AKI, particularly when platelet count is incorporated within the scoring system 170 . Another study demonstrated the use of prognostic biomarkers for diagnostic and predictive enrichment in paediatric SA-AKI 171 . However, notable differences remain between current recommendations of management of SA-AKI for adult and paediatric patients, particularly with regard to fluid management and the type of fluid, with a preference for the use of balanced crystalloids in children. The aforementioned fluid recommendations in this manuscript apply to adults specifically 172 . Of note, a 2021 study reported the inclusion of paediatric populations in the study of EBP therapies to treat SA-AKI via a selective cytopheretic device 173 . Future work should align paediatric-specific sepsis definitions with SA-AKI management and research agendas (Box 6 ). A Society of Critical Care Medicine (SCCM) task force is currently working to streamline a definition of paediatric sepsis, but, for now, a precise definition of paediatric SA-AKI remains unavailable.
Box 6 SA-AKI in paediatric patients
Consensus statement 6a
Development, as a biological variable, might affect the pathophysiology and management of SA-AKI across the lifespan (not graded).
Consensus statement 6b
Neonates and children merit consideration and inclusion in SA-AKI research (not graded).
How should SA-AKI be defined in the paediatric population?
Are there differences in the pathophysiology of SA-AKI across the lifespan?
How can the proposed research agenda incorporate the concept of development as a biological variable in the diagnosis and management of SA-AKI?
Conclusions
The presence of AKI in patients with sepsis is common and SA-AKI is best defined by both consensus sepsis criteria and AKI criteria, with early SA-AKI occurring within 48 h of diagnosis of sepsis and late SA-AKI occurring between 48 h and 7 days of diagnosis of sepsis. Multiple mechanisms can contribute to the development of SA-AKI and their relative contributions might define distinct SA-AKI endotypes. These endotypes might be identified through the use of biomarkers, including functional, stress and tissue damage-related biomarkers, as well as clinical information. Prognostic information should help to determine treatment, which should follow currently accepted guidelines, but the use of specific therapies might be influenced by the endotype. For example, the SA-AKI endotype might affect the choice of vasopressor or dictate whether EBP techniques might be used for immunomodulatory support in patients who meet explicit criteria.
Singer, M. et al. The Third International Consensus definitions for sepsis and septic shock (Sepsis-3). JAMA 315 , 801–810 (2016).
Article CAS PubMed PubMed Central Google Scholar
Uchino, S. et al. Acute renal failure in critically ill patients: a multinational, multicenter study. JAMA 294 , 813–818 (2005).
Article CAS PubMed Google Scholar
Hoste, E. A. et al. Epidemiology of acute kidney injury in critically ill patients: the multinational AKI-EPI study. Intensive Care Med. 41 , 1411–1423 (2015).
Article PubMed Google Scholar
Peerapornratana, S., Manrique-Caballero, C. L., Gomez, H. & Kellum, J. A. Acute kidney injury from sepsis: current concepts, epidemiology, pathophysiology, prevention and treatment. Kidney Int. 96 , 1083–1099 (2019).
Article PubMed PubMed Central Google Scholar
Poston, J. T. & Koyner, J. L. Sepsis associated acute kidney injury. BMJ 364 , k4891 (2019).
Schuler, A. et al. The impact of acute organ dysfunction on long-term survival in Sepsis. Crit. Care Med. 46 , 843–849 (2018).
Stanski, N. L. et al. Severe acute kidney injury is independently associated with mortality in children with septic shock. Intensive Care Med. 46 , 1050–1051 (2020).
Zarbock, A., Gomez, H. & Kellum, J. A. Sepsis-induced acute kidney injury revisited: pathophysiology, prevention and future therapies. Curr. Opin. Crit. Care 20 , 588–595 (2014).
Kaddourah, A., Basu, R. K., Bagshaw, S. M., Goldstein, S. L. & Investigators, A. Epidemiology of acute kidney injury in critically ill children and young adults. N. Engl. J. Med. 376 , 11–20 (2017).
Wiersema, R. et al. Two subphenotypes of septic acute kidney injury are associated with different 90-day mortality and renal recovery. Crit. Care 24 , 150 (2020).
Basu, R. K. et al. Clinical phenotypes of acute kidney injury are associated with unique outcomes in critically ill septic children. Pediatr. Res. 90 , 1031–1038 (2021).
Kellum, J. A., Bellomo, R. & Ronco, C. Acute Dialysis Quality Initiative (ADQI): methodology. Int. J. Artif. Organs 31 , 90–93 (2008).
Nadim, M. K. et al. Cardiac and vascular surgery-associated acute kidney injury: the 20th International Consensus Conference of the ADQI (Acute Disease Quality Initiative) Group. J. Am. Heart Assoc. https://doi.org/10.1161/JAHA.118.008834 (2018).
Alonso-Coello, P. et al. GRADE Evidence to Decision (EtD) frameworks: a systematic and transparent approach to making well informed healthcare choices. 1: introduction. BMJ 353 , i2016 (2016).
Liu, J., Xie, H., Ye, Z., Li, F. & Wang, L. Rates, predictors, and mortality of sepsis-associated acute kidney injury: a systematic review and meta-analysis. BMC Nephrol. 21 , 318 (2020).
Kellum, J. A. & Lameire, N., KDIGO AKI Guideline Work Group Diagnosis, evaluation, and management of acute kidney injury: a KDIGO summary (Part 1). Crit. Care 17 , 204 (2013).
Mehta, R. L. et al. Sepsis as a cause and consequence of acute kidney injury: program to improve care in acute renal disease. Intensive Care Med. 37 , 241–248 (2011).
Lotvall, J. et al. Asthma endotypes: a new approach to classification of disease entities within the asthma syndrome. J. Allergy Clin. Immunol. 127 , 355–360 (2011).
Seymour, C. W. et al. Precision medicine for all? Challenges and opportunities for a precision medicine approach to critical illness. Crit. Care 21 , 257 (2017).
Bhatraju, P. K. et al. Acute kidney injury subphenotypes based on creatinine trajectory identifies patients at increased risk of death. Crit. Care 20 , 372 (2016).
Kellum, J. A., Sileanu, F. E., Bihorac, A., Hoste, E. A. & Chawla, L. S. Recovery after acute kidney injury. Am. J. Respir. Crit. Care Med. 195 , 784–791 (2017).
Lima, R. S. et al. Comparison between early and delayed acute kidney injury secondary to infectious disease in the intensive care unit. Int. Urol. Nephrol. 40 , 731–739 (2008).
Bagshaw, S. M., George, C., Bellomo, R. & Committee, A. D. M. Early acute kidney injury and sepsis: a multicentre evaluation. Crit. Care 12 , R47 (2008).
Bagshaw, S. M. et al. Septic acute kidney injury in critically ill patients: clinical characteristics and outcomes. Clin. J. Am. Soc. Nephrol. 2 , 431–439 (2007).
Vincent, J. L. et al. Sepsis in European intensive care units: results of the SOAP study. Crit. Care Med. 34 , 344–353 (2006).
Cruz, D. N. et al. North east Italian prospective hospital renal outcome survey on acute kidney injury (NEiPHROS-AKI): targeting the problem with the RIFLE criteria. Clin. J. Am. Soc. Nephrol. 2 , 418–425 (2007).
Kolhe, N. V., Stevens, P. E., Crowe, A. V., Lipkin, G. W. & Harrison, D. A. Case mix, outcome and activity for patients with severe acute kidney injury during the first 24 hours after admission to an adult, general critical care unit: application of predictive models from a secondary analysis of the ICNARC Case Mix Programme database. Crit. Care 12 (Suppl 1), S2 (2008).
Xu, K. et al. Unique transcriptional programs identify subtypes of AKI. J. Am. Soc. Nephrol. 28 , 1729–1740 (2017).
Bhatraju, P. K. et al. Identification of acute kidney injury subphenotypes with differing molecular signatures and responses to vasopressin therapy. Am. J. Respir. Crit. Care Med. 199 , 863–872 (2019).
Treszl, A. et al. Interleukin genetic variants and the risk of renal failure in infants with infection. Pediatr. Nephrol. 17 , 713–717 (2002).
Gordon, A. C. et al. TNF and TNFR polymorphisms in severe sepsis and septic shock: a prospective multicentre study. Genes Immun. 5 , 631–640 (2004).
Jaber, B. L. et al. Cytokine gene promoter polymorphisms and mortality in acute renal failure. Cytokine 25 , 212–219 (2004).
Wattanathum, A., Manocha, S., Groshaus, H., Russell, J. A. & Walley, K. R. Interleukin-10 haplotype associated with increased mortality in critically ill patients with sepsis from pneumonia but not in patients with extrapulmonary sepsis. Chest 128 , 1690–1698 (2005).
Cardinal-Fernandez, P. et al. Genetic predisposition to acute kidney injury induced by severe sepsis. J. Crit. Care 28 , 365–370 (2013).
Frank, A. J. et al. BCL2 genetic variants are associated with acute kidney injury in septic shock*. Crit. Care Med. 40 , 2116–2123 (2012).
Vilander, L. M., Kaunisto, M. A., Vaara, S. T. & Pettila, V., group, F. s. Genetic variants in SERPINA4 and SERPINA5, but not BCL2 and SIK3 are associated with acute kidney injury in critically ill patients with septic shock. Crit. Care 21 , 47 (2017).
Lu, J. C. et al. Searching for genes that matter in acute kidney injury: a systematic review. Clin. J. Am. Soc. Nephrol. 4 , 1020–1031 (2009).
Vilander, L. M., Kaunisto, M. A. & Pettila, V. Genetic predisposition to acute kidney injury — a systematic review. BMC Nephrol. 16 , 197 (2015).
Cardinal-Fernandez, P. et al. [Genetic determinants of acute renal damage risk and prognosis: a systematic review]. Med. Intensiv. 36 , 626–633 (2012).
CAS Google Scholar
Allis, C. D. & Jenuwein, T. The molecular hallmarks of epigenetic control. Nat. Rev. Genet. 17 , 487–500 (2016).
Lehner, M. D., Morath, S., Michelsen, K. S., Schumann, R. R. & Hartung, T. Induction of cross-tolerance by lipopolysaccharide and highly purified lipoteichoic acid via different Toll-like receptors independent of paracrine mediators. J. Immunol. 166 , 5161–5167 (2001).
Hato, T. et al. The macrophage mediates the renoprotective effects of endotoxin preconditioning. J. Am. Soc. Nephrol. 26 , 1347–1362 (2015).
He, K. et al. Lipopolysaccharide-induced cross-tolerance against renal ischemia-reperfusion injury is mediated by hypoxia-inducible factor-2α-regulated nitric oxide production. Kidney Int. 85 , 276–288 (2014).
Zager, R. A. ‘Biologic memory’ in response to acute kidney injury: cytoresistance, Toll-like receptor hyper-responsiveness and the onset of progressive renal disease. Nephrol. Dial. Transplant. 28 , 1985–1993 (2013).
Zager, R. A., Johnson, A. C., Lund, S. & Hanson, S. Acute renal failure: determinants and characteristics of the injury-induced hyperinflammatory response. Am. J. Physiol. Renal Physiol. 291 , F546–F556 (2006).
Naito, M., Bomsztyk, K. & Zager, R. A. Endotoxin mediates recruitment of RNA polymerase II to target genes in acute renal failure. J. Am. Soc. Nephrol. 19 , 1321–1330 (2008).
Naito, M., Zager, R. A. & Bomsztyk, K. BRG1 increases transcription of proinflammatory genes in renal ischemia. J. Am. Soc. Nephrol. 20 , 1787–1796 (2009).
Medzhitov, R., Schneider, D. S. & Soares, M. P. Disease tolerance as a defense strategy. Science 335 , 936–941 (2012).
Ayres, J. S. & Schneider, D. S. Tolerance of infections. Annu. Rev. Immunol. 30 , 271–294 (2012).
Ferreira, A. et al. Sickle hemoglobin confers tolerance to Plasmodium infection. Cell 145 , 398–409 (2011).
Ramos, S. et al. Renal control of disease tolerance to malaria. Proc. Natl Acad. Sci. USA 116 , 5681–5686 (2019).
Larsen, R. et al. A central role for free heme in the pathogenesis of severe sepsis. Sci. Transl. Med. 2 , 51ra71 (2010).
Jin, K. et al. Activation of AMP-activated protein kinase during sepsis/inflammation improves survival by preserving cellular metabolic fitness. FASEB J. 34 , 7036–7057 (2020).
Toro, J., Manrique-Caballero, C. L. & Gomez, H. Metabolic reprogramming and host tolerance: a novel concept to understand sepsis-associated AKI. J. Clin. Med. https://doi.org/10.3390/jcm10184184 (2021).
Wang, A. et al. Opposing effects of fasting metabolism on tissue tolerance in bacterial and viral inflammation. Cell 166 , 1512–1525 e1512 (2016).
Basile, D. P., Leonard, E. C., Tonade, D., Friedrich, J. L. & Goenka, S. Distinct effects on long-term function of injured and contralateral kidneys following unilateral renal ischemia-reperfusion. Am. J. Physiol. Renal Physiol. 302 , F625–F635 (2012).
Grgic, I. et al. Targeted proximal tubule injury triggers interstitial fibrosis and glomerulosclerosis. Kidney Int. 82 , 172–183 (2012).
Basile, D. P., Donohoe, D., Roethe, K. & Osborn, J. L. Renal ischemic injury results in permanent damage to peritubular capillaries and influences long-term function. Am. J. Physiol. Renal Physiol. 281 , F887–F899 (2001).
Pagtalunan, M. E., Olson, J. L., Tilney, N. L. & Meyer, T. W. Late consequences of acute ischemic injury to a solitary kidney. J. Am. Soc. Nephrol. 10 , 366–373 (1999).
Sharma, A., Mucino, M. J. & Ronco, C. Renal functional reserve and renal recovery after acute kidney injury. Nephron Clin. Pract. 127 , 94–100 (2014).
Jacob, K. A. et al. Intraoperative high-dose dexamethasone and severe AKI after cardiac surgery. J. Am. Soc. Nephrol. 26 , 2947–2951 (2015).
Pickkers, P. et al. Effect of human recombinant alkaline phosphatase on 7-day creatinine clearance in patients with sepsis-associated acute kidney injury: a randomized clinical trial. JAMA 320 , 1998–2009 (2018).
Tumlin, J. A. et al. Outcomes in patients with vasodilatory shock and renal replacement therapy treated with intravenous angiotensin II. Crit. Care Med. 46 , 949–957 (2018).
Zhou, C. et al. Levosimendan for prevention of acute kidney injury after cardiac surgery: a meta-analysis of randomized controlled trials. Am. J. Kidney Dis. 67 , 408–416 (2016).
Tholen, M., Ricksten, S. E. & Lannemyr, L. Effects of levosimendan on renal blood flow and glomerular filtration in patients with acute kidney injury after cardiac surgery: a double blind, randomized placebo-controlled study. Crit. Care 25 , 207 (2021).
Swaminathan, M. et al. Allogeneic mesenchymal stem cells for treatment of AKI after cardiac surgery. J. Am. Soc. Nephrol. 29 , 260–267 (2018).
Himmelfarb, J. et al. Perioperative THR-184 and AKI after cardiac surgery. J. Am. Soc. Nephrol. 29 , 670–679 (2018).
Bromberg, J. S. et al. Renal function improvement following ANG-3777 treatment in patients at high risk for delayed graft function after kidney transplantation. Transplantation 105 , 443–450 (2021).
Evans, L. et al. Surviving Sepsis campaign: international guidelines for management of sepsis and septic shock 2021. Crit. Care Med. 49 , e1063–e1143 (2021).
Kellum, J. A., Mythen, M. G. & Shaw, A. D. The 12th Consensus Conference of the Acute Dialysis Quality Initiative (ADQI XII). Br. J. Anaesth. 113 , 729–731 (2014).
Chen, K. P. et al. Peripheral edema, central venous pressure, and risk of AKI in critical illness. Clin. J. Am. Soc. Nephrol. 11 , 602–608 (2016).
Legrand, M. et al. Association between systemic hemodynamics and septic acute kidney injury in critically ill patients: a retrospective observational study. Crit. Care 17 , R278 (2013).
Eskesen, T. G., Wetterslev, M. & Perner, A. Systematic review including re-analyses of 1148 individual data sets of central venous pressure as a predictor of fluid responsiveness. Intensive Care Med. 42 , 324–332 (2016).
Payen, D. et al. A positive fluid balance is associated with a worse outcome in patients with acute renal failure. Crit. Care 12 , R74 (2008).
Garzotto, F. et al. The dose response multicentre investigation on fluid assessment (DoReMIFA) in critically ill patients. Crit. Care 20 , 196 (2016).
Douglas, I. S. et al. Fluid response evaluation in sepsis hypotension and shock: a randomized clinical trial. Chest 158 , 1431–1445 (2020).
Woodcock, T. E. & Woodcock, T. M. Revised Starling equation and the glycocalyx model of transvascular fluid exchange: an improved paradigm for prescribing intravenous fluid therapy. Br. J. Anaesth. 108 , 384–394 (2012).
Milford, E. M. & Reade, M. C. Resuscitation fluid choices to preserve the endothelial glycocalyx. Crit. Care 23 , 77 (2019).
Byrne, L. et al. Unintended consequences: fluid resuscitation worsens shock in an ovine model of endotoxemia. Am. J. Respir. Crit. Care Med. 198 , 1043–1054 (2018).
Self, W. H. et al. Liberal versus restrictive intravenous fluid therapy for early septic shock: rationale for a randomized trial. Ann. Emerg. Med. 72 , 457–466 (2018).
Keijzers, G. et al. The Australasian resuscitation in sepsis evaluation: FLUid or vasopressors in emergency department Sepsis, a multicentre observational study (ARISE FLUIDS observational study): rationale, methods and analysis plan. Emerg. Med. Australas. 31 , 90–96 (2019).
Vaara, S. T. et al. Restrictive fluid management versus usual care in acute kidney injury (REVERSE-AKI): a pilot randomized controlled feasibility trial. Intensive Care Med. 47 , 665–673 (2021).
Meyhoff, T. S. et al. Restriction of intravenous fluid in ICU patients with septic shock. N. Engl. J. Med. 386 , 2459–2470 (2022).
Zampieri, F. G. et al. Effect of intravenous fluid treatment with a balanced solution vs 0.9% saline solution on mortality in critically ill patients: the BaSICS randomized clinical trial. JAMA https://doi.org/10.1001/jama.2021.11684 (2021).
Finfer, S. et al. A comparison of albumin and saline for fluid resuscitation in the intensive care unit. N. Engl. J. Med. 350 , 2247–2256 (2004).
Beran, A. et al. Balanced crystalloids versus normal saline in adults with sepsis: a comprehensive systematic review and meta-analysis. J. Clin. Med. https://doi.org/10.3390/jcm11071971 (2022).
Hammond, D. A. et al. Balanced crystalloids versus saline in critically ill adults: a systematic review and meta-analysis. Ann. Pharmacother. 54 , 5–13 (2020).
Semler, M. W. et al. Balanced crystalloids versus saline in critically ill adults. N. Engl. J. Med. 378 , 829–839 (2018).
Self, W. H. et al. Balanced crystalloids versus saline in noncritically ill adults. N. Engl. J. Med. 378 , 819–828 (2018).
Caironi, P. et al. Albumin replacement in patients with severe sepsis or septic shock. N. Engl. J. Med. 370 , 1412–1421 (2014).
Lewis, S. R. et al. Colloids versus crystalloids for fluid resuscitation in critically ill people. Cochrane Database Syst. Rev. 8 , CD000567 (2018).
PubMed Google Scholar
Sakr, Y. et al. Randomized controlled multicentre study of albumin replacement therapy in septic shock (ARISS): protocol for a randomized controlled trial. Trials 21 , 1002 (2020).
Perner, A. et al. Hydroxyethyl starch 130/0.42 versus Ringer’s acetate in severe sepsis. N. Engl. J. Med. 367 , 124–134 (2012).
Moeller, C. et al. How safe is gelatin? A systematic review and meta-analysis of gelatin-containing plasma expanders vs crystalloids and albumin. J. Crit. Care. 35 , 75–83 (2016).
Ragaller, M. J., Theilen, H. & Koch, T. Volume replacement in critically ill patients with acute renal failure. J. Am. Soc. Nephrol. 12 (Suppl 17), S33–S39 (2001).
Dickenmann, M., Oettl, T. & Mihatsch, M. J. Osmotic nephrosis: acute kidney injury with accumulation of proximal tubular lysosomes due to administration of exogenous solutes. Am. J. Kidney Dis. 51 , 491–503 (2008).
Jaber, S. et al. Sodium bicarbonate therapy for patients with severe metabolic acidaemia in the intensive care unit (BICAR-ICU): a multicentre, open-label, randomised controlled, phase 3 trial. Lancet 392 , 31–40 (2018).
Russell, J. A. et al. Vasopressin versus norepinephrine infusion in patients with septic shock. N. Engl. J. Med. 358 , 877–887 (2008).
Wichmann, S. et al. Loop diuretics in adult intensive care patients with fluid overload: a systematic review of randomised clinical trials with meta-analysis and trial sequential analysis. Ann. Intensive Care 12 , 52 (2022).
Berthelsen, R. E. et al. Forced fluid removal in intensive care patients with acute kidney injury: the randomised FFAKI feasibility trial. Acta Anaesthesiol. Scand. 62 , 936–944 (2018).
Silversides, J. A. et al. Feasibility of conservative fluid administration and deresuscitation compared with usual care in critical illness: the role of active deresuscitation after resuscitation-2 (RADAR-2) randomised clinical trial. Intensive Care Med. 48 , 190–200 (2022).
Silbert, B. I. et al. Determinants of urinary output response to IV furosemide in acute kidney injury: a pharmacokinetic/pharmacodynamic study. Crit. Care Med. 44 , e923–e929 (2016).
Khanna, A., Ostermann, M. & Bellomo, R. Angiotensin II for the treatment of vasodilatory shock. N. Engl. J. Med. 377 , 2604 (2017).
Ostermann, M. et al. Recommendations on acute kidney injury biomarkers from the acute disease quality initiative consensus conference: a consensus statement. JAMA Netw. Open 3 , e2019209 (2020).
Molinari, L. et al. Utility of biomarkers for sepsis-associated acute kidney injury staging. JAMA Netw. Open 5 , e2212709 (2022).
Depret, F. et al. Incidence and outcome of subclinical acute kidney injury using penKid in critically ill patients. Am. J. Respir. Crit. Care Med. 202 , 822–829 (2020).
Hollinger, A. et al. Proenkephalin A 119-159 (Penkid) is an early biomarker of septic acute kidney injury: the kidney in sepsis and septic shock (Kid-SSS) study. Kidney Int. Rep. 3 , 1424–1433 (2018).
Martensson, J., Martling, C. R., Oldner, A. & Bell, M. Impact of sepsis on levels of plasma cystatin C in AKI and non-AKI patients. Nephrol. Dial. Transplant. 27 , 576–581 (2012).
Frazee, E. et al. Cystatin C-guided vancomycin dosing in critically ill patients: a quality improvement project. Am. J. Kidney Dis. 69 , 658–666 (2017).
Peters, B. J. et al. Impact of serum cystatin C-based glomerular filtration rate estimates on drug dose selection in hospitalized patients. Pharmacotherapy 38 , 1068–1073 (2018).
Kashani, K. et al. Discovery and validation of cell cycle arrest biomarkers in human acute kidney injury. Crit. Care 17 , R25 (2013).
Bagshaw, S. M. et al. Plasma and urine neutrophil gelatinase-associated lipocalin in septic versus non-septic acute kidney injury in critical illness. Intensive Care Med. 36 , 452–461 (2010).
de Geus, H. R., Betjes, M. G., Schaick, R. & Groeneveld, J. A. Plasma NGAL similarly predicts acute kidney injury in sepsis and nonsepsis. Biomark. Med. 7 , 415–421 (2013).
Basu, R. K. et al. Incorporation of biomarkers with the renal angina index for prediction of severe AKI in critically ill children. Clin. J. Am. Soc. Nephrol. 9 , 654–662 (2014).
Honore, P. M. et al. Urinary tissue inhibitor of metalloproteinase-2 and insulin-like growth factor-binding protein 7 for risk stratification of acute kidney injury in patients with sepsis. Crit. Care Med. 44 , 1851–1860 (2016).
R, D. S. & D, C. S. Recent advances in bedside device-based early detection of sepsis. J. Intensive Care Med. 37 , 849–856 (2022).
Article Google Scholar
Sinha, P., Churpek, M. M. & Calfee, C. S. Machine learning classifier models can identify acute respiratory distress syndrome phenotypes using readily available clinical data. Am. J. Respir. Crit. Care Med. 202 , 996–1004 (2020).
Bhatraju, P. K. et al. Genetic variation implicates plasma angiopoietin-2 in the development of acute kidney injury sub-phenotypes. BMC Nephrol. 21 , 284 (2020).
Knox, D. B., Lanspa, M. J., Kuttler, K. G., Brewer, S. C. & Brown, S. M. Phenotypic clusters within sepsis-associated multiple organ dysfunction syndrome. Intensive Care Med. 41 , 814–822 (2015).
Chaudhary, K. et al. Utilization of deep learning for subphenotype identification in sepsis-associated acute kidney injury. Clin. J. Am. Soc. Nephrol. 15 , 1557–1565 (2020).
Kwong, Y. D. et al. Using best subset regression to identify clinical characteristics and biomarkers associated with sepsis-associated acute kidney injury. Am. J. Physiol. Renal Physiol. 319 , F979–F987 (2020).
Chawla, L. S. et al. Acute kidney disease and renal recovery: consensus report of the Acute Disease Quality Initiative (ADQI) 16 workgroup. Nat. Rev. Nephrol. 13 , 241–257 (2017).
Bhatraju, P. K. et al. Association between early recovery of kidney function after acute kidney injury and long-term clinical outcomes. JAMA Netw. Open 3 , e202682 (2020).
Siew, E. D. et al. Timing of recovery from moderate to severe AKI and the risk for future loss of kidney function. Am. J. Kidney Dis. 75 , 204–213 (2020).
Uhel, F. et al. Mortality and host response aberrations associated with transient and persistent acute kidney injury in critically ill patients with sepsis: a prospective cohort study. Intensive Care Med. 46 , 1576–1589 (2020).
Macedo, E. et al. Quality of care after AKI development in the hospital: consensus from the 22nd Acute Disease Quality Initiative (ADQI) conference. Eur. J. Intern. Med. 80 , 45–53 (2020).
Karsanji, D. J. et al. Disparity between nephrologists’ opinions and contemporary practices for community follow-up after AKI hospitalization. Clin. J. Am. Soc. Nephrol. 12 , 1753–1761 (2017).
Menon, S. et al. Urinary biomarker incorporation into the renal angina index early in intensive care unit admission optimizes acute kidney injury prediction in critically ill children: a prospective cohort study. Nephrol. Dial. Transplant. 31 , 586–594 (2016).
Darmon, M., Truche, A. S., Abdel-Nabey, M., Schnell, D. & Souweine, B. Early recognition of persistent acute kidney injury. Semin. Nephrol. 39 , 431–441 (2019).
Peerapornratana, S. et al. Sepsis-associated acute kidney disease. Kidney Int. Rep. 5 , 839–850 (2020).
Cutuli, S. L., Carelli, S., Grieco, D. L. & De Pascale, G. Immune modulation in critically ill septic patients. Medicina https://doi.org/10.3390/medicina57060552 (2021).
Jarczak, D., Kluge, S. & Nierhaus, A. Sepsis-pathophysiology and therapeutic concepts. Front. Med. 8 , 628302 (2021).
Rimmele, T. & Kellum, J. A. Clinical review: blood purification for sepsis. Crit. Care 15 , 205 (2011).
Girardot, T., Schneider, A. & Rimmele, T. Blood purification techniques for sepsis and septic AKI. Semin. Nephrol. 39 , 505–514 (2019).
Husain-Syed, F. et al. Extracorporeal organ support (ECOS) in critical illness and acute kidney injury: from native to artificial organ crosstalk. Intensive Care Med. 44 , 1447–1459 (2018).
Romagnoli, S., Ricci, Z. & Ronco, C. CRRT for sepsis-induced acute kidney injury. Curr. Opin. Crit. Care 24 , 483–492 (2018).
Zarbock, A. et al. Effect of early vs delayed initiation of renal replacement therapy on mortality in critically ill patients with acute kidney injury: the ELAIN randomized clinical trial. JAMA 315 , 2190–2199 (2016).
Gaudry, S. et al. Initiation strategies for renal-replacement therapy in the intensive care unit. N. Engl. J. Med. 375 , 122–133 (2016).
Investigators, S.-A. et al. Timing of initiation of renal-replacement therapy in acute kidney injury. N. Engl. J. Med. 383 , 240–251 (2020).
Barbar, S. D. et al. Timing of renal-replacement therapy in patients with acute kidney injury and sepsis. N. Engl. J. Med. 379 , 1431–1442 (2018).
Chen, W. Y. et al. The timing of continuous renal replacement therapy initiation in sepsis-associated acute kidney injury in the intensive care unit: the CRTSAKI Study (Continuous RRT Timing in Sepsis-associated AKI in ICU): study protocol for a multicentre, randomised controlled trial. BMJ Open 11 , e040718 (2021).
Martin-Loeches, I. et al. Surviving sepsis campaign: research opportunities for infection and blood purification therapies. Crit. Care Explor. 3 , e0511 (2021).
Benichou, N. et al. Vascular access for renal replacement therapy among 459 critically ill patients: a pragmatic analysis of the randomized AKIKI trial. Ann. Intensive Care 11 , 56 (2021).
Ronco, C. & Reis, T. Continuous renal replacement therapy and extended indications. Semin. Dial. 34 , 550–560 (2021).
Stockmann, H. et al. CytoSorb rescue for COVID-19 patients with vasoplegic shock and multiple organ failure: a prospective, open-label, randomized controlled pilot study. Crit. Care Med. 50 , 964–976 (2022).
Diab, M. et al. Cytokine hemoadsorption during cardiac surgery versus standard surgical care for infective endocarditis (REMOVE): results from a multicenter randomized controlled trial. Circulation 145 , 959–968 (2022).
Ikeda, T., Ikeda, K., Suda, S. & Ueno, T. Usefulness of the endotoxin activity assay as a biomarker to assess the severity of endotoxemia in critically ill patients. Innate Immun. 20 , 881–887 (2014).
Kellum, J. A., Foster, D. & Walker, P. M. Endotoxemic shock: a molecular phenotype in sepsis. Nephron https://doi.org/10.1159/000525548 (2022).
Biagioni, E. et al. Endotoxin activity levels as a prediction tool for risk of deterioration in patients with sepsis not admitted to the intensive care unit: a pilot observational study. J. Crit. Care 28 , 612–617 (2013).
Romaschin, A. D., Klein, D. J. & Marshall, J. C. Bench-to-bedside review: clinical experience with the endotoxin activity assay. Crit. Care 16 , 248 (2012).
Lee, W. Y., Kim, H. J. & Kim, E. Y. Impact of polymyxin B hemoperfusion therapy on high endotoxin activity level patients after successful infection source control: a prospective cohort study. Sci. Rep. 11 , 24132 (2021).
Klein, D. J. et al. Polymyxin B hemoperfusion in endotoxemic septic shock patients without extreme endotoxemia: a post hoc analysis of the EUPHRATES trial. Intensive Care Med. 44 , 2205–2212 (2018).
Payen, D. M. et al. Early use of polymyxin B hemoperfusion in patients with septic shock due to peritonitis: a multicenter randomized control trial. Intensive Care Med. 41 , 975–984 (2015).
Ronco, C. et al. Extracorporeal therapies in non-renal disease: treatment of sepsis and the peak concentration hypothesis. Blood Purif. 22 , 164–174 (2004).
Ronco, C. & Bellomo, R. Hemoperfusion: technical aspects and state of the art. Crit. Care 26 , 135 (2022).
Berger, M. M. et al. Nutrients and micronutrients at risk during renal replacement therapy: a scoping review. Curr. Opin. Crit. Care 27 , 367–377 (2021).
Shaw, A. R. & Mueller, B. A. Antibiotic dosing in continuous renal replacement therapy. Adv. Chronic Kidney Dis. 24 , 219–227 (2017).
de Geus, H. R. H., Smeets, T., Hoek, R. A. S., Endeman, H. & Hunfeld, N. The Seraph-100 microbind affinity blood filter does not affect vancomycin, tacrolimus, and mycophenolic acid plasma concentrations. Blood Purif. 50 , 971–975 (2021).
Schmidt, J. J., Eden, G., Seffer, M. T., Winkler, M. & Kielstein, J. T. In vitro elimination of anti-infective drugs by the Seraph® 100 Microbind® affinity blood filter. Clin. Kidney J. 13 , 421–424 (2020).
CAS PubMed PubMed Central Google Scholar
Godi, I. et al. Vancomycin adsorption during in vitro model of hemoperfusion with HA380 cartridge. Nephron 145 , 157–163 (2021).
Shimokawa, K. et al. Adsorption of various antimicrobial agents to endotoxin removal polymyxin-B immobilized fiber (Toraymyxin®). Colloids Surf. B Biointerfaces 90 , 58–61 (2012).
Liebchen, U. et al. No clinically relevant removal of meropenem by cytokine adsorber CytoSorb® in critically ill patients with sepsis or septic shock. Intensive Care Med. 47 , 1332–1333 (2021).
Konig, C. et al. In vitro removal of anti-infective agents by a novel cytokine adsorbent system. Int. J. Artif. Organs 42 , 57–64 (2019).
Rhee, H. et al. The role of the specialized team in the operation of continuous renal replacement therapy: a single-center experience. BMC Nephrol. 18 , 332 (2017).
Joannes-Boyau, O., Velly, L. & Ichai, C. Optimizing continuous renal replacement therapy in the ICU: a team strategy. Curr. Opin. Crit. Care 24 , 476–482 (2018).
Neyra, J. A. & Goldstein, S. L. Optimizing renal replacement therapy deliverables through multidisciplinary work in the intensive care unit. Clin. Nephrol. 90 , 1–5 (2018).
de Grooth, H. J., Parienti, J. J. & Oudemans-van Straaten, H. M. Should we rely on trials with disease- rather than patient-oriented endpoints? Intensive Care Med. 44 , 464–466 (2018).
Weiss, S. L. et al. Global epidemiology of pediatric severe sepsis: the sepsis prevalence, outcomes, and therapies study. Am. J. Respir. Crit. Care Med. 191 , 1147–1157 (2015).
Goldstein, S. L. et al. Consensus-based recommendations on priority activities to address acute kidney injury in children: a modified Delphi consensus statement. JAMA Netw. Open 5 , e2229442 (2022).
Stanski, N. L. et al. Recalibration of the renal angina index for pediatric septic shock. Kidney Int. Rep. 6 , 1858–1867 (2021).
Stanski, N. L. et al. PERSEVERE biomarkers predict severe acute kidney injury and renal recovery in pediatric septic shock. Am. J. Respir. Crit. Care Med. 201 , 848–855 (2020).
Weiss, S. L. et al. Surviving sepsis campaign international guidelines for the management of septic shock and sepsis-associated organ dysfunction in children. Intensive Care Med. 46 , 10–67 (2020).
Goldstein, S. L. et al. Use of the selective cytopheretic device in critically ill children. Kidney Int. Rep. 6 , 775–784 (2021).
Ismail, O. Z. et al. Kidney injury molecule-1 protects against Gα12 activation and tissue damage in renal ischemia-reperfusion injury. Am. J. Pathol. 185 , 1207–1215 (2015).
Stevens, P. E., Levin A.; Kidney Disease: Improving Global Outcomes Chronic Kidney Disease Guideline Development Work Group Members. KDIGO 2012 clinical practice guideline for the evaluation and management of chronic kidney disease. Kidney Int. Suppl. 3 , 1–150 (2013).
Google Scholar
Bagshaw, S. M. et al. Urinary biomarkers in septic acute kidney injury. Intensive Care Med. 33 , 1285–1296 (2007).
Kamijo-Ikemori, A. et al. [Urinary L-type fatty acid binding protein (L-FABP) as a new urinary biomarker promulgated by the Ministry of Health, Labour and Welfare in Japan]. Rinsho. Byori. 61 , 635–640 (2013).
CAS PubMed Google Scholar
de Geus, H. R., Bakker, J., Lesaffre, E. M. & le Noble, J. L. Neutrophil gelatinase-associated lipocalin at ICU admission predicts for acute kidney injury in adult patients. Am. J. Respir. Crit. Care Med. 183 , 907–914 (2011).
Palsson, R. et al. Assessment of interobserver reliability of nephrologist examination of urine sediment. JAMA Netw. Open 3 , e2013959 (2020).
Bagshaw, S. M. et al. A prospective evaluation of urine microscopy in septic and non-septic acute kidney injury. Nephrol. Dial. Transplant. 27 , 582–588 (2012).
Toh, L., Bitker, L., Eastwood, G. M. & Bellomo, R. The incidence, characteristics, outcomes and associations of small short-term point-of-care creatinine increases in critically ill patients. J. Crit. Care 52 , 227–232 (2019).
Rewa, O. G. et al. The furosemide stress test for prediction of worsening acute kidney injury in critically ill patients: a multicenter, prospective, observational study. J. Crit. Care 52 , 109–114 (2019).
Husain-Syed, F. et al. Congestive nephropathy: a neglected entity? Proposal for diagnostic criteria and future perspectives. ESC Heart Fail. 8 , 183–203 (2021).
Abdelhafez, M. et al. Diagnostic performance of fractional excretion of sodium for the differential diagnosis of acute kidney injury: a systematic review and meta-analysis. Clin. J. Am. Soc. Nephrol. 17 , 785–797 (2022).
Basu, R. K., Kaddourah, A., Goldstein, S. L. & Investigators, A. S. Assessment of a renal angina index for prediction of severe acute kidney injury in critically ill children: a multicentre, multinational, prospective observational study. Lancet Child Adolesc. Health 2 , 112–120 (2018).
Tverring, J. et al. Heparin-binding protein (HBP) improves prediction of sepsis-related acute kidney injury. Ann. Intensive Care 7 , 105 (2017).
Ragan, D., Horvath-Szalai, Z., Szirmay, B. & Muhl, D. Novel damage biomarkers of sepsis-related acute kidney injury. EJIFCC 33 , 11–22 (2022).
Hu, Q. et al. Association between admission serum procalcitonin and the occurrence of acute kidney injury in patients with septic shock: a retrospective cohort study. Sci. Prog. 104 , 368504211043768 (2021).
Shiao, C. C., Chueh, Y. F., Yang, L. & Nsarf Using procalcitonin to predict acute kidney injury in septic patients: caveat emptor? J. Formos. Med. Assoc. 118 , 542–544 (2019).
de Werra, I. et al. Cytokines, nitrite/nitrate, soluble tumor necrosis factor receptors, and procalcitonin concentrations: comparisons in patients with septic shock, cardiogenic shock, and bacterial pneumonia. Crit. Care Med. 25 , 607–613 (1997).
Iglesias, J., Marik, P. E., Levine, J. S. & Norasept, I. I. S. I. Elevated serum levels of the type I and type II receptors for tumor necrosis factor-α as predictive factors for ARF in patients with septic shock. Am. J. Kidney Dis. 41 , 62–75 (2003).
Su, L. X. et al. Diagnostic value of urine sTREM-1 for sepsis and relevant acute kidney injuries: a prospective study. Crit. Care 15 , R250 (2011).
Maslove, D. M. et al. Redefining critical illness. Nat. Med. 28 , 1141–1148 (2022).
Download references
Acknowledgements
The authors wish to acknowledge Ms. Gioia Vencato and Annamaria Saccardo, for their help in organizing this ADQI meeting. This conference was kindly supported by unrestricted educational grants from AM Pharma, Baxter, Biomerieux, Cytosorbants, Exthera Medical, Jafron, Ortho Clinical Diagnostics, Paion, Spectral and Sphingotec GmbH.
Author information
These authors contributed equally: Alexander Zarbock, Mitra K. Nadim.
Authors and Affiliations
Department of Anaesthesiology, Intensive Care and Pain Medicine, University Hospital of Münster, Münster, Germany
Alexander Zarbock & Melanie Meersch
Outcomes Research Consortium, Cleveland, OH, USA
Alexander Zarbock
Division of Nephrology and Hypertension, Department of Medicine, Keck School of Medicine, University of Southern California, Los Angeles, CA, USA
Mitra K. Nadim
Dept Intensive Care Medicine, Radboud University Medical Centre, Nijmegen, The Netherlands
Peter Pickkers
Program for Critical Care Nephrology, Department of Critical Care Medicine, University of Pittsburgh, Pittsburgh, PA, USA
Hernando Gomez
Division of Population Health and Genomics, University of Dundee, Dundee, UK
Samira Bell
Division of Intensive Care and Emergency Medicine, Department of Internal Medicine, Medical University Innsbruck, Innsbruck, Austria
Michael Joannidis
Division of Nephrology and Hypertension, Division of Pulmonary and Critical Care Medicine, Department of Medicine, Mayo Clinic, Rochester, MN, USA
Kianoush Kashani
Department of Medicine, University of Chicago, Chicago, IL, USA
Jay L. Koyner
Department of Medicine, University of Alberta, Edmonton, Alberta, Canada
Neesh Pannu
D’Or Institute for Research and Education (IDOR), Division of Kidney Transplantation, DF Star Hospital, SGAS 914, Asa Sul, 70390-140, Brasília, Brazil
Thiago Reis
Laboratory of Molecular Pharmacology, Faculty of Health Sciences, University of Brasília, Asa Norte, Campus Darcy Ribeiro, 70910-900, Brasília, Brazil
Anaesthesiology and Critical Care Medicine, Edouard Herriot Hospital, Hospices Civils de Lyon, Lyon, France
Thomas Rimmelé
Department of Critical Care Medicine, Faculty of Medicine and Dentistry, University of Alberta and Alberta Health Services, Edmonton, Alberta, Canada
- Sean M. Bagshaw
Department of Critical Care, University of Melbourne, Parkville, Australia
Rinaldo Bellomo & Emily J. See
Department of Intensive Care, Austin Hospital, Melbourne, Victoria, Australia
Rinaldo Bellomo
Australian and New Zealand Intensive Care Research Centre, Monash University, Melbourne, Australia
Department of Intensive Care, Royal Melbourne Hospital, Melbourne, Australia
Nephrology and Kidney Transplantation Unit, Department of Translational Medicine, University of Piemonte Orientale (UPO), “Maggiore della Carità” University Hospital, Novara, Italy
Vicenzo Cantaluppi
Paediatric Intensive Care Unit, King’s College Hospital NHS Foundation Trust, London, UK
Centre for Medical Sciences - CISMed, University of Trento, Trento, Italy
Silvia De Rosa
Anaesthesia and Intensive Care, Santa Chiara Regional Hospital, APSS, Trento, Italy
Servei de Medicina Intensiva, Hospital Universitari de Bellvitge, L’Hospitalet de Llobregat, Barcelona, Spain
Xose Perez-Fernandez
Department of Internal Medicine II, University Hospital Giessen and Marburg, Justus-Liebig-University Giessen, Giessen, Germany
Faeq Husain-Syed
Department of Pharmacy and Therapeutics, School of Pharmacy, University of Pittsburgh, Pittsburgh, PA, USA
Sandra L. Kane-Gill
Department of Critical Care, Tallaght University Hospital, Tallaght, Dublin, Ireland
Yvelynne Kelly
School of Medicine, Trinity College Dublin, Dublin, Ireland
Department of Medicine, University of California San Diego, La Jolla, CA, USA
Ravindra L. Mehta
School of Medicine, University College Dublin, Dublin, Ireland
Patrick T. Murray
Department of Intensive Care, King’s College London, Guy’s & St Thomas’ Hospital, London, UK
- Marlies Ostermann
William Harvey Research Institute, Faculty of Medicine and Dentistry, Queen Mary University of London, London, UK
John Prowle
Department of Anaesthesia and Critical Care, Meyer Children’s University Hospital, Florence, Italy
Zaccaria Ricci
Department of Health Sciences, Section of Anaesthesiology and Intensive Care, University of Florence, Florence, Italy
Department of Nephrology, Royal Melbourne Hospital, Melbourne, Victoria, Australia
Emily J. See
Adult Intensive Care Unit, Centre Hospitalier Universitaire Vaudois (CHUV), Lausanne, Switzerland
Antoine Schneider
Department of Paediatrics, Indiana University School of Medicine, Indianapolis, IN, USA
Danielle E. Soranno
Department of Medicine, University of Alabama at Birmingham, Birmingham, AL, USA
Ashita Tolwani
Department of Health Sciences, Section of Anaesthesiology, Intensive Care and Pain Medicine. University of Florence. Azienda Ospedaliero Universitaria Careggi, Florence, Italy
Gianluca Villa
Department of Medicine, University of Padova, Padua, Italy
Claudio Ronco
International Renal Research Institute of Vicenza (IRRV), Vicenza, Italy
Department of Nephrology, San Bortolo Hospital, Vicenza, Italy
Department of Critical Care, Royal Surrey Hospital Foundation Trust, Guildford, Surrey, UK
Lui G. Forni
Faculty of Health Sciences, University of Surrey, Guildford, Surrey, UK
You can also search for this author in PubMed Google Scholar
Contributions
All authors made equal contributions to the discussion of the content and researching data for the article. Members of each group contributed equally to writing their sections. L.G.F., A.Z. and M.K.N. combined the workgroup drafts and edited for style and length. All authors reviewed the final manuscript before submission.
Corresponding author
Correspondence to Lui G. Forni .
Ethics declarations
Competing interests.
A.Z. has received consulting fees from Astute-bioMérieux, Baxter, Bayer, Novartis, Guard Therapeutics, AM Pharma, Paion, Fresenius, research funding from Astute-bioMérieux, Fresenius, Baxter, and speakers fees from Astute-bioMérieux, Fresenius, Baxter; P.P. has received speaker’s honoraria/travel/consultancy reimbursements as a member of an advisory board or steering committee from Baxter, EBI, AM-Pharma, Sphingotec, Adrenomed, 4Teen4, and Paion; H.G. serves as scientific adviser for Novartis and Trilinear bioventures, and has received research grants from bioMérieux, Baxter and TES Pharma; M.J. has received honoraria and research support from Baxter Healthcare Corp, AM-Pharma, CLS Behring, Fresenius and Novartis; K.K. has received research grants from Philips Research North America and Google, speaker’s honorarium from Nikkiso Critical Care Medical Supplies (Shanghai) Co., Ltd, funding from National Institute of Diabetes and Digestive and Kidney Diseases grant (R01DK131586) and consulting fees from Baxter Inc. to Mayo Clinic; J.L.K. has received consulting fees from Astute-bioMérieux, Sphingotec, Pfizer, Baxter, Mallinckrodt, Novartis, Guard Therapeutics, research funding from Astute-bioMérieux Medical, Bioporto, NxStage, Fresenius, Satellite Healthcare, and speakers fees from NxStage medical; M.M. received lecture fees from bioMérieux, Fresenius Medical Care and Baxter; T. Reis has received funding for lectures, been consultant or advisory board member for AstraZeneca, B. Braun, Baxter, bioMérieux, Boehringer Ingelheim, Contatti Medical (CytoSorbents), Eurofarma, Fresenius Medical Care, Jafron, Lifepharma and Nova Biomedical; T. Rimmelé serves as a scientific adviser for Jafron and Exthera, has received funding for lectures from B. Braun, Baxter, bioMérieux, Exthera, Fresenius Medical Care, Estor and Jafron, and has received research grants from Baxter and Fresenius Medical Care; S.M.B. is supported by a Canada Research Chair in Critical Care Outcomes and Systems Evaluation; R.B. has received grant money, speaker’s fees and advisory board fees from Baxter Acute Care, Jafron Biomedical, CSL Behring, AM Pharma and Paion; V.C. has received lecture fees from Baxter, Estor-Toray and Aferetica-Cytosorbents; S.L.K.-G. is an elected member of the Executive Committee (or Council) of the Society of Critical Care Medicine (SCCM) (the views presented are those of the author and do not represent the views of SCCM); R.L.M. has consulting/advisory relationships with Baxter, AM Pharma, bioMérieux, Intercept, Mallinckrodt, GE Healthcare, Medtronic, CHF Solutions, Sphingotec, Abiomed, Nova Biomed, Sanofi, Renasym, Alexion, Fresenius, Abbott and Renibus; P.T.M. serves as a scientific adviser for AM-Pharma, Novartis, and Renibus Therapeutics; M.O. has received speaker honoraria from Fresenius Medical, Baxter and bioMérieux, and research funding from Fresenius Medical, Baxter and bioMérieux; J.P. has received research support from Jafron Biomedical Co Ltd and bioMérieux SA, and consultancy or lecture fees from Baxter Inc, Nikkiso Europe GmbH, Mission Therapeutics Ltd and Paion UK Ltd; A.S. has received speaker and/or consulting honoraria from Fresenius Medical Care, CytoSorbents SA, Jafron, Medtronics and B. Braun Avitum, and has received grants from the Leenaards foundation and B Braun Melsungen; A.T. has received consulting fees from Baxter and royalties from 0.5% citrate patent from Baxter; G.V. received lecture fees from Baxter; C.R. has been on the advisory boards or speaker’s bureau for Asahi, Aferetica, Baxter, bioMérieux, Cytosorbents, B. Braun, GE, Medica, Medtronic, Jafron and AstraZeneca; L.G.F. has received research support and lecture fees from Ortho Clinical Diagnostics, Baxter, Exthera and bioMérieux, and consulting fees from La Jolla Pharmaceuticals and Paion; the remaining authors declare no competing interests.
Peer review
Peer review information.
Nature Reviews Nephrology thanks M. Bestle, K. Doi, D. Ponce and R. Raina for their contribution to the peer review of this work.
Additional information
Publisher’s note Springer Nature remains neutral with regard to jurisdictional claims in published maps and institutional affiliations.
Related links
ADQI: www.adqi.org
Supplementary information
Supplementary information, rights and permissions.
Springer Nature or its licensor (e.g. a society or other partner) holds exclusive rights to this article under a publishing agreement with the author(s) or other rightsholder(s); author self-archiving of the accepted manuscript version of this article is solely governed by the terms of such publishing agreement and applicable law.
Reprints and permissions
About this article
Cite this article.
Zarbock, A., Nadim, M.K., Pickkers, P. et al. Sepsis-associated acute kidney injury: consensus report of the 28th Acute Disease Quality Initiative workgroup. Nat Rev Nephrol 19 , 401–417 (2023). https://doi.org/10.1038/s41581-023-00683-3
Download citation
Accepted : 18 January 2023
Published : 23 February 2023
Issue Date : June 2023
DOI : https://doi.org/10.1038/s41581-023-00683-3
Share this article
Anyone you share the following link with will be able to read this content:
Sorry, a shareable link is not currently available for this article.
Provided by the Springer Nature SharedIt content-sharing initiative
This article is cited by
Sepsis-associated acute kidney injury: recent advances in enrichment strategies, sub-phenotyping and clinical trials.
- Matthieu Legrand
Critical Care (2024)
Identifying hub genes of sepsis-associated and hepatic encephalopathies based on bioinformatic analysis—focus on the two common encephalopathies of septic cirrhotic patients in ICU
BMC Medical Genomics (2024)
The influence of gender on the epidemiology of and outcome from sepsis associated acute kidney injury in ICU: a retrospective propensity-matched cohort study
- Ruiqiang Zheng
European Journal of Medical Research (2024)
The pathophysiology of sepsis and precision-medicine-based immunotherapy
- Evangelos J. Giamarellos-Bourboulis
- Anna C. Aschenbrenner
- Mihai G. Netea
Nature Immunology (2024)
Acute kidney injury in patients with burns
- Audra T. Clark
Nature Reviews Nephrology (2024)
Quick links
- Explore articles by subject
- Guide to authors
- Editorial policies
Sign up for the Nature Briefing newsletter — what matters in science, free to your inbox daily.

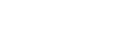
- Advanced Life Support
- Endocrinology
- Gastroenterology
- Infectious disease
- Intensive care
- Palliative Care
- Respiratory
- Rheumatology
- Haematology
- Endocrine surgery
- General surgery
- Neurosurgery
- Ophthalmology
- Plastic surgery
- Vascular surgery
- Abdo examination
- Cardio examination
- Neurolo examination
- Resp examination
- Rheum examination
- Vasc exacmination
- Other examinations
- Clinical Cases
- Communication skills
- Prescribing
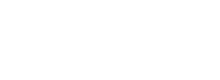
Acute kidney injury case study with questions and answers
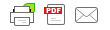
Single best answer question based on renal clinical case:
Single best answer question 1.
- A 50 year old alcoholic male presents with sepsis secondary to klebsiella pneumonia. His background includes IHD, previous pneumonia, hypercholesterolaemia and hypertension. Medications include: furosemide, enalapril, aspirin, clopidogrel, co-amoxiclav (current) and simvastatin
- He is treated with IV antibiotics and is managed on an ITU setting for 1 week
- Potassium 5.0
- Urea 24 (from 8)
- Creatinine 390 (from 60)
- Clinically he is mildly dry, with a BP 135/83, HR 90, he is catheterised with a U/O 35ml/hr
Which one of the following is the best management option?
- Switch to high dose IV furosemide, stop enalapril, give IV fluids to maintain urine output, daily bloods
- Stop furosemide, stop enalapril, add in dopamine and maintain adequate hydration to maintain urine output, daily bloods
- Stop furosemide, stop enalapril, adequate fluids to maintain urine output, daily bloods
- Continue furosemide, stop enalapril, high dose corticosteroids and continue adequate fluids to maintain urine output, daily bloods
- None of the above
- This man has risk factors for AKI (hypertension and dehydration) and is on various renotoxic medications including aspirin, enalapril (ACE-I) and furosemide (loop diuretic).
- The key is to maintain hydration to keep urine output reasonable (>0.5ml/kg/hr) while stopping as many renotoxic medications as possible.
- Fluids should be given and frusemide and enalapril stopped. He has a history of ischaemic heart disease so stopping aspirin is not ideal. Daily bloods to monitor response is advised.
- There is no evidence for dopamine (mentioned in option 2) or hydrocortisone (mentioned in option 4).
- Open access
- Published: 03 September 2023
Acute kidney injury in neurocritical care
- Faeq Husain-Syed 1 , 2 na1 ,
- Tomonori Takeuchi 3 , 4 na1 ,
- Javier A. Neyra 3 ,
- Gonzalo Ramírez-Guerrero 5 , 6 , 7 ,
- Mitchell H. Rosner 1 ,
- Claudio Ronco 8 , 9 &
- Ashita J. Tolwani 3
Critical Care volume 27 , Article number: 341 ( 2023 ) Cite this article
11k Accesses
3 Citations
58 Altmetric
Metrics details
Approximately 20% of patients with acute brain injury (ABI) also experience acute kidney injury (AKI), which worsens their outcomes. The metabolic and inflammatory changes associated with AKI likely contribute to prolonged brain injury and edema. As a result, recognizing its presence is important for effectively managing ABI and its sequelae. This review discusses the occurrence and effects of AKI in critically ill adults with neurological conditions, outlines potential mechanisms connecting AKI and ABI progression, and highlights AKI management principles. Tailored approaches include optimizing blood pressure, managing intracranial pressure, adjusting medication dosages, and assessing the type of administered fluids. Preventive measures include avoiding nephrotoxic drugs, improving hemodynamic and fluid balance, and addressing coexisting AKI syndromes. ABI patients undergoing renal replacement therapy (RRT) are more susceptible to neurological complications. RRT can negatively impact cerebral blood flow, intracranial pressure, and brain tissue oxygenation, with effects tied to specific RRT methods. Continuous RRT is favored for better hemodynamic stability and lower risk of dialysis disequilibrium syndrome. Potential RRT modifications for ABI patients include adjusted dialysate and blood flow rates, osmotherapy, and alternate anticoagulation methods. Future research should explore whether these strategies enhance outcomes and if using novel AKI biomarkers can mitigate AKI-related complications in ABI patients.
Graphical abstract
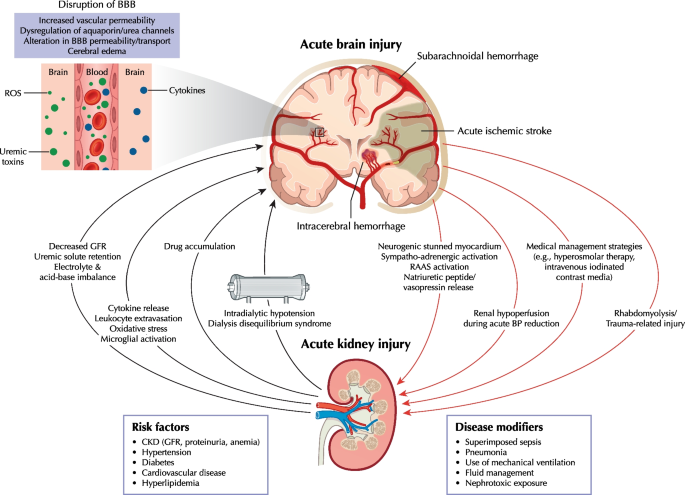
Acute kidney injury (AKI) in intensive care units (ICUs) is an independent risk factor for death. Reported mortality rates from AKI with renal replacement therapy (RRT; 40–55%) are higher than the mortality rates due to ICU-related myocardial infarction (20%), sepsis without AKI (15–25%), or acute respiratory distress syndrome requiring mechanical ventilation (30–40%) [ 1 ]. This high mortality rate is attributed to the systemic impact of AKI on the brain, heart, lungs, liver, and gastrointestinal tract, linking AKI to various syndromes (Additional file 1 ) [ 2 ]. Beyond the acute phase, AKI increases the likelihood of chronic kidney disease (CKD), cardiovascular complications, recurrent AKI, and functional impairment [ 3 ].
In the context of neurocritical care, AKI diagnosis holds significance for acute brain injury (ABI) management. This article summarizes the epidemiology and outcomes of AKI in neurocritically ill adults admitted to the ICU, focusing on prevalent ABI conditions like traumatic brain injury (TBI), aneurysmal subarachnoid hemorrhage (SAH), intracerebral hemorrhage (ICH), and acute stroke. The review explores potential AKI mechanisms and their involvement in reciprocal ABI progression, while also highlighting essential principles in AKI management. Additionally, considerations for managing RRT in the context of ABI are discussed. Our literature search strategy is provided in Additional file 2 .
AKI epidemiology and outcomes in neurocritical care
Studies on AKI prevalence in neurocritical care are few, and their results vary with AKI definition [ 4 , 5 , 6 ] (Additional file 3 ). Current AKI diagnosis and classification are based on the 2012 KDIGO ( Kidney Disease: Improving Global Outcomes ) consensus criteria [ 6 ] (Additional file 4 ; left panel). This three-stage severity classification system is based on changes in serum creatinine (SCr) concentration and urine output (UOP). However, most neurocritical care studies only used SCr-based definitions because SCr data are readily available in electronic health records, missing a substantial proportion of patients with AKI [ 7 ].
In a multinational study spanning 97 ICUs and encompassing more than 1800 patients, with 25.9% primarily diagnosed with neurological symptoms, 57% of the entire cohort developed AKI by KDIGO criteria within the initial week of admission [ 8 ]. Among these, 39% experienced stages 2–3 AKI, and 13.5% required RRT, accounting for 23.5% of AKI cases [ 8 ]. While insights into AKI incidence among neurocritical patients mainly stem from retrospective studies in single centers and exclude cases diagnosed with AKI upon admission, a recent study in a mixed neurological/neurosurgical ICU reported an overall AKI occurrence of 23.5% utilizing the SCr/UOP KDIGO criteria [ 9 ]. Interestingly, similar AKI rates were noted among specific brain pathologies, including TBI, 11.9–26.7% (KDIGO, SCr only) [ 10 , 11 , 12 , 13 , 14 ] and 17.7% (SCr/UOP KDIGO) [ 15 ]; acute stroke, 20.9–43.3% (KDIGO, SCr only) [ 16 , 17 ]; non-traumatic aneurysmal SAH, 16.7–23.7% (KDIGO, SCr only) [ 18 , 19 ], and ICH, 20.0–29.9% [ 18 , 20 , 21 , 22 ]. The few studies assessing RRT in patients with ABI reported overall rates of 0.3–5.6% among neurocritical patients [ 17 , 23 ]. Irrespective of the criteria employed and the specific brain condition under consideration, AKI severity is associated with increased mortality, obstacles to rehabilitation care, increased likelihood of disability at discharge, and potential downstream effects on healthcare services [ 8 , 10 , 24 , 25 , 26 , 27 ].
Data on renal recovery after AKI post-ABI are scarce [ 18 ], and long-term renal outcomes after AKI in ABI patients remain unknown.
AKI causes and pathophysiological mechanisms
Clinicians must consider various AKI causes specific to both neurocritical and non-neurological ICU patients (Additional file 1 ). The Graphical Abstract summarizes key brain-kidney interaction pathways in neurocritical care that could lead to reciprocal ABI and AKI progression.
CKD plays a significant role as a risk factor for AKI in neurocritical patients, with those having CKD displaying a fivefold higher prevalence of cerebrovascular disease compared to those without CKD [ 10 , 26 , 28 ]. These data are unsurprising, given that the kidneys and brain share common vascular risk factors, including hypertension, diabetes, and hyperlipidemia. Notably, proteinuria of ≥ 30 mg/dL and CKD stages 3–4 are established risk factors for cerebrovascular disease, which, in turn, increases the likelihood of AKI [ 29 , 30 ]. A study on patients with spontaneous ICH found that individuals with CKD experience worse functional outcomes (OR 1.91; 95% CI 1.04–3.52) and higher mortality (OR 3.33; 95% CI 1.76–6.27) at 12 months post-ICH compared to those without CKD [ 31 ]. While TBI patients tend to be younger and possess normal pre-existing renal function, patients with acute stroke, aneurysmal SAH, and ICH are generally older and more likely to have underlying CKD.
ABI can be complicated by neurogenic stunned myocardium [ 32 ], which is a transient and diffuse form of left ventricular cardiomyopathy, likely triggered by autonomic nervous system activation with excess catecholamine release, impaired myocardial glucose metabolism secondary to neurocardiogenic injury, and coronary microvascular dysfunction [ 33 , 34 ]. Patients with this condition exhibit a wide range of cardiac abnormalities including arrhythmias and ventricular dysfunction [ 33 , 34 ], increasing the risk of AKI due to reduced cardiac output, pulmonary edema, and prolonged vasopressor support [ 35 ]. However, the prevalence of AKI in such patients has not been described. Autonomic nervous system imbalance also contributes to sympatho-adrenergic drive and renin–angiotensin–aldosterone system activation and vasopressin release, resulting in renal vasoconstriction, enhanced sodium and water reabsorption, and decreased renal blood flow and glomerular filtration rate (GFR) [ 36 ]. Neurogenic stunned myocardium prevalence in patients with SAH is high and estimated at ~ 30% [ 32 ].
The 2020 Neurocritical Care Society (NCS) guidelines suggest, with low evidence, using hyperosmolar therapy for initial management of cerebral edema in neurocritical patients with SAH, TBI, acute ischemic stroke, and ICH [ 37 ]. While mannitol is the most frequently administered hyperosmolar solution, its use, particularly at high doses, has been associated with an increased risk of AKI in such patients [ 38 , 39 , 40 ]. Risk factors for mannitol-associated AKI include higher illness severity, heart failure, diabetes, use of diuretics, and lower baseline GFR [ 38 , 41 ]. The precise mechanism for mannitol-associated AKI is not well defined but may be related to increased serum osmolality [ 42 ] and appears to be dose-related [ 11 ]. The 2020 NCS guidelines recommend hypertonic sodium solution over mannitol as it might be more effective in reducing ICP or cerebral edema ABI [ 37 ]; however, its usage can lead to hypernatremia and hyperchloremia, both of which have been associated with an increased AKI risk [ 19 , 43 , 44 ]. However, the effects of high chloride content on the kidneys have been debated [ 45 , 46 ]. While experimental studies suggest it causes renal vasoconstriction and decreased GFR [ 47 , 48 , 49 ], a post-hoc analyses of the EPO-TBI and COBI trials demonstrated no association between hypertonic sodium solution and AKI [ 11 , 14 ]. Notably, randomized controlled studies specifically focusing on AKI and acid–base balance outcomes related to hyperosmolar therapy in neurocritical care are lacking.
Intravenous contrast might be a concern in patients with ABI due to increased AKI risk, particularly in patients with SAH who might receive substantial amounts of contrast due to repeated computed tomography angiography scans for the diagnosis and/or management of cerebral vasospasm/delayed cerebral ischemia. However, existing evidence suggests that the risks associated with low- or iso-osmolar intravenous contrast agents are minimal, and AKI is infrequent in patients with eGFR of ≥ 30 mL/min/1.73 m 2 [ 50 , 51 ]. Intraarterial contrast administration poses a greater risk for AKI because such procedures often require larger doses that reach the renal arteries at high concentrations and potentially lead to atheroembolic complications [ 52 ].
Sepsis and nosocomial infections, especially ventilator-associated pneumonia, are common in neurocritical patients. A retrospective single-center study reported sepsis (75%) and respiratory infections (68%) as the main non-neurological complications in patients with TBI [ 53 ]. Although data on patients with ABI are limited, infectious complications are considered the main AKI disease modifiers during ICU stay [ 16 , 26 ], in line with general ICU populations [ 8 ].
Myoglobin-associated rhabdomyolysis and renal toxicity should be considered in patients with TBI. A recent multicenter registry study found rhabdomyolysis present in 3.8% of patients with TBI and independently associated with AKI occurrence [ 54 ]. Moreover, while rhabdomyolysis and elevated serum/urinary myoglobin correlate with AKI occurrence, they also correlate with trauma severity and other renal insults. Hence, the direct contribution of rhabdomyolysis to kidney injury is often uncertain. The causative role of myoglobin toxicity in AKI is more certain in cases with severe rhabdomyolysis (creatinine kinase > 15,000 IU/L), which rarely occurs in patients with TBI.
Brain injury progression following AKI
Experimental evidence suggests that while AKI is an indicator of illness severity, it can cause further organ dysfunction and damage. Despite limitations due to interspecies differences, animal kidney injury models have been used extensively to elucidate the mechanisms leading to remote organ dysfunction after AKI (Table 1 ).
AKI causes systemic inflammation by generating proinflammatory cytokines and reducing cytokine clearance [ 63 , 64 ]. These inflammatory processes contribute to AKI initiation and likely perpetuate and extend brain injury. Consequently, the brain and kidneys might interact during AKI by amplifying cytokine-induced damage and oxidative stress, extravasating leukocytes, and dysregulating cerebral aquaporin channels [ 65 ]. The various proinflammatory and metabolic changes observed in AKI (e.g., waste solute retention, disturbances of inorganic solute metabolism, and reduced drug clearance) can disrupt the blood–brain barrier. This disruption may lead to an influx of water and the accumulation of inflammatory cells, cytokines, and neurotoxic substances in the central nervous system. This accumulation can result in cerebral edema, inflammation, hemorrhage, and even death [ 66 ]. Animal models have supported this mechanism, demonstrating blood–brain barrier disruption [ 59 ], microvascular protein leakage, microglial cell activation [ 59 ], hippocampal injury, inflammation characterized by neuronal pyknosis [ 62 ], and increased soluble inflammatory proteins in the cerebral cortex and hippocampus [ 59 ] following renal ischemia–reperfusion injury-induced AKI. Importantly, neuronal pyknosis did not increase in corresponding animal models of acute liver injury, suggesting that some observed effects are relatively specific to AKI and not associated with generalized inflammation following acute organ injury [ 59 ]. Functionally, mice with AKI showed impaired locomotor function that correlated with renal ischemia duration [ 59 ]. Alterations in neurotransmitter secretion and uptake during AKI might worsen brain injury and dysfunction [ 66 ]. Furthermore, sepsis and liver failure, which are frequently observed in critical illness, can exacerbate brain injury and/or AKI and contribute to multiple organ dysfunction [ 67 ].
AKI management
The underlying cause of AKI should be identified promptly, paying special attention to reversible causes. AKI-related syndromes (Additional file 1 ) pose a major challenge to AKI management, so regular re-evaluation is required for adaptive management. Since current evidence does not suggest that AKI in neurocritical patients should be managed differently from AKI in other critically ill populations, KDIGO-bundle recommendations (i.e., reduce nephrotoxic agents, monitor SCr/UOP, discontinue renin-angiotensin-system blockers, optimize fluid status) are considered appropriate [ 68 ]. No externally-validated scoring system is available to evaluate AKI risk in neurocritical patients.
Hemodynamic management
Blood pressure (BP) targets should consider premorbid BP. The potential benefits of increased renal perfusion must be weighed against potentially deleterious effects on cerebral perfusion. Cerebral autoregulation is impaired in approximately one-third of patients with TBI [ 69 ], in whom a rise in mean arterial pressure (MAP) might increase the ICP due to hyperemia, while a drop in MAP might lead to cerebral hypoperfusion. Although targeting high MAP (≥ 80 mmHg) in patients with sepsis and chronic hypertension could benefit renal outcomes [ 70 ], careful evaluation of its effects on ICP and, consequently, on cerebral perfusion pressure (CPP) is required. As disease-specific data are lacking, CPP targets for ABI are usually derived from TBI guidelines, which recommend maintaining CPP at 60–70 mmHg and assessing cerebral autoregulation to individualized CPP targets [ 71 , 72 ].
Norepinephrine is the first-line vasopressor used in sepsis with organ dysfunction and ABI [ 73 , 74 ]. Data suggest that vasopressin could benefit some sepsis-associated AKI subtypes, but its role in patients with ABI and AKI is not fully known [ 75 , 76 ]. Notably, vasopressin should be used cautiously as it might increase the risk of hyponatremia (and subsequent cerebral edema).
The magnitude of acute systolic blood pressure (SBP) reduction in patients with ICH, aimed at limiting hematoma growth, requires careful monitoring. According to the 2022 guidelines from the American Heart Association/American Stroke Association, reducing acute SBP to 140 mmHg and maintaining it within the range of 130–150 mmHg is regarded as safe and potentially beneficial for enhancing functional outcomes in patients with mild-to-moderate ICH who initially present with an SBP between 150–220 mmHg [ 77 ]. Because chronic hypertension shifts the plateau of the renal autoregulatory curve to higher levels [ 78 ], a sudden decrease in blood pressure (BP) could lead to significantly compromised tissue perfusion. A targeted stepwise BP reduction rather than absolute targeted value could optimize renal perfusion and mitigate AKI. In a post-hoc analysis of ATACH-II, which included patients with initial systolic blood pressure (SBP) of ≥ 220 mmHg (22.8% of the group), a significant reduction in SBP (110–139 mmHg) resulted in a higher rate of neurological dysfunction at 24 h and more renal complications by the seventh day of discharge [ 79 ]. This was compared to standard SBP lowering (140–179 mmHg), without any benefits in reducing hematoma growth at 24 h or rates of death or severe disability at 90 days [ 79 ]. This suggests that cautious lowering of blood pressure might be necessary for this specific subgroup. Subsequent analysis of ATACH-II indicated that a baseline SCr ≥ 1.25 mg/dL and higher intravenous nicardipine doses were associated with increased risk for AKI [ 80 ]. Accordingly, another study on patients with ICH indicated that an intensive SBP reduction to > 90 mmHg in the first 12 h increases the risk of AKI regardless of preexisting CKD [ 21 ].
Fluid management
Much of the evidence on fluid management in ABI is derived from TBI guidelines. A negative fluid balance has been associated with adverse outcomes in patients with TBI [ 81 ], whereas fluid overload can cause systemic complications or cerebral edema and increased ICP [ 81 , 82 ]. A multicenter study evaluating variable fluid management in patients with TBI found that incrementally positive fluid balance was associated with increased ICU mortality (OR, 1.10 per 0.1 L increase; 95% CI 1.07–1.12) and poor functional outcomes (OR, 1.04 per 0.1 L increase; 95% CI 1.02–1.05) [ 83 ]. Notably, these data likely represent confounding by indication, as sicker patients are more likely to receive additional volume. While the study did not assess renal outcomes, patients receiving a mean daily fluid balance of ≥ 0.37 L were more likely to undergo RRT than those with < 0.37 L (4% vs. 2%; P = 0.021) [ 83 ]. Reports on the association between fluid balance and renal outcomes in ABI are scarce. However, in non-neurological critically ill patients, the relationship between fluid overload and AKI is well-established due to factors like venous congestion, increased renal interstitial pressure, and decreased renal blood flow and GFR as observed in patients with cardiorenal syndrome [ 84 ].
The 2018 ESICM ( European Society of Intensive Care Medicine ) consensus statement recommends using MAP, fluid balance, and multimodal monitoring (e.g., ICP, brain tissue oxygen tension, autoregulatory status) to optimize fluid therapy in neurocritically ill patients [ 85 ]. Point-of-care focused ultrasonography is increasingly used to determine fluid status rapidly, facilitating personalized fluid management when appropriate [ 86 ]; however, their role in the neurocritical care setting has not been defined.
Several studies have focused on the administered fluid type. Current data do not conclusively support routine use of balanced crystalloid solutions over 0.9% saline to reduce the risk of AKI and RRT in critically ill patients. A recent meta-analysis showed that the risk ratios of developing AKI and of being treated with RRT with balanced crystalloids compared with 0.9% saline were 0.96 (95% CI 0.89–1.02) and 0.95 (95% CI 0.81–1.11), respectively [ 87 ]. However, the 2018 ESICM consensus statement recommends crystalloids as maintenance and resuscitation fluids in neurocritical care patients while not recommending albumin and hypotonic solutions [ 85 ]. Small single-center randomized trials in patients with SAH [ 88 ] and TBI [ 89 ] found that balanced crystalloids reduced the hyperchloremia rate compared to 0.9% saline. Synthetic colloids (e.g., starch, gelatin) should be avoided in patients with ABI as they increase the risk of AKI and death [ 85 , 90 ].
Hyperosmolar therapy
Hypernatremia and hyperchloremia are common complications in patients with ABI and risk factors for AKI and excess mortality [ 18 , 19 ]. Consequently, fluid choice should be informed by the need to correct the specific electrolyte and acid–base imbalances. SCr and UOP should be closely monitored in patients receiving hyperosmolar therapy due to its strong association with AKI occurrence [ 6 , 37 ]. The 2020 NCS guidelines recommend an upper serum sodium range of 150–155 mmol/L and chloride range of 110–115 mmol/L to decrease the risk of AKI In patients receiving hypertonic sodium solutions [ 37 ]. Bolus administration could be considered over continuous hypertonic sodium solution infusion as it could lead to fewer chloride values aberrations [ 20 , 91 ]. An osmolality threshold of ≥ 320 mOsm/L has been suggested to increase the risk of AKI in patients receiving mannitol infusion [ 42 ]; however, this threshold has recently been questioned [ 41 ].
Nephrotoxic exposure and drug dosing
Medications should be closely reviewed for potentially nephrotoxic agents, which should be discontinued or substituted with less nephrotoxic drugs. However, potentially nephrotoxic agents, e.g., intravenous contrast, should still be used in patients with ABI if the information gained could have important therapeutic implications. The 2020 American College of Radiology and National Kidney Foundation guidelines recommend prophylactic 0.9% saline before and after intravenous contrast exposure in patients with eGFR < 30 mL/min/1.73 m 2 or recent AKI to reduce the risk of AKI [ 50 ]. Drug-induced acute interstitial nephritis (e.g., due to anticonvulsants or antibiotics) must also be considered [ 92 , 93 ].
Augmented renal clearance of > 130 mL/min/1.73 m 2 is common in patients with ABI. The 74% (95% CI 55–87) pooled prevalence of augmented renal clearance in neurocritical care patients reported in a recent meta-analysis is higher than in any other critical care population [ 94 ]. Postulated mechanisms that promote an augmented renal clearance in ABI patients include increased cardiac output, high serum atrial natriuretic peptides, and increased hypothalamus–pituitary–adrenal axis activity with elevated levels of cortisol and catecholamines [ 94 , 95 ]. Augmented renal clearance has important implications for drug dosing in patients with ABI and may lead to underdosing of levetiracetam and vancomycin [ 96 , 97 ]; therefore, clinicians should monitor renal-eliminated medications to achieve target trough concentrations.
Biomarkers for AKI risk assessment
Factors contributing to AKI development and progression could be modified by incorporating novel biomarkers of early tubular stress or damage when clinical interventions or exposures increase the risk of AKI progression. Whether early identification of these patients could help reduce ABI-associated morbidity remains to be determined. A recent Acute Dialysis Quality Initiative consensus statement suggested that the AKI definition should be augmented by integrating novel AKI biomarkers into its risk-classification (Additional file 4 ; right panel) [ 98 ]. Although many candidate biomarkers exist (Additional file 5 ), prospective validation and implementation are needed. Among the few biomarkers studied in neurocritical care, cystatin C, neutrophil gelatinase-associated lipocalin, and liver-type fatty acid-binding protein at admission have been associated with increased risk of AKI in patients with TBI or stroke [ 99 , 100 , 101 , 102 ].
- Renal replacement therapy
Patients with ABI may require RRT to manage the consequences of impaired renal function, including electrolyte imbalances, metabolic acidosis, and fluid overload. However, the use of RRT can impact cerebral blood flow, CPP, ICP, and brain tissue oxygenation, potentially leading to neurological complications. The effect on these factors depends on the specific type of RRT and its outcomes, which calls for special consideration in ABI patients [ 103 ].
Effects of RRT on the brain
RRT might lead to exacerbation of cerebral edema through the “reverse urea effect” or dialysis disequilibrium syndrome (DDS). During the initial stages of RRT, effective osmolytes such as urea are rapidly removed from the blood, creating an osmotic gradient between the blood and brain tissue. As brain cells have a relatively slow transport rate through cell membranes, this gradient causes water to move into the brain tissue, resulting in cerebral edema [ 104 ]. Additionally, elevated bicarbonate levels in dialysate and rapid rise in pH may induce paradoxical intracellular acidosis. This phenomenon occurs when bicarbonate-derived carbon dioxide crosses the cell membrane, leading to neuronal swelling and cerebral edema [ 105 ]. DDS and cerebral edema could lead to brain herniation or decreased CPP in patients with ABI.
Intradialytic hypotension, defined as a decrease in SBP by ≥ 20 mmHg or a reduction in MAP by ≥ 10 mmHg with associated symptoms [ 106 ], might occur during RRT and affect CPP because BP is its key determinant. It occurs when the body's hemodynamic compensation mechanisms fail to respond adequately to the decrease in plasma volume caused by ultrafiltration. This leads to reduced cardiac output, impaired peripheral vasoconstriction and refilling capacity, and decreased MAP [ 107 ]. If cerebral autoregulation is impaired, excessive ultrafiltration with decreased MAP might lead to reduced cerebral perfusion and CPP, potentially resulting in decreased ICP.
RRT has the potential to reduce brain tissue oxygenation through multiple pathways. One mechanism involves dialysis-related brain edema, which can impede oxygen diffusion. Additionally, increased ICP associated with a hypermetabolic state in the brain may increase oxygen consumption [ 108 ]. Dialysis-induced inflammation could result in pulmonary leukosequestration, leading to reduced arterial oxygen levels. This can impact the brain's respiratory center perfusion and metabolism, ultimately decreasing cerebral oxygenation and causing intermittent short apneic episodes [ 109 ]. In a recent study involving 17 adult hemodialysis patients, magnetic resonance imaging, diffusion tensor imaging, and proton magnetic resonance spectroscopy demonstrated that a single hemodialysis session could lead to an increase in brain tissue volume during the session [ 110 ]. This change was accompanied by alterations in white matter diffusion metrics and brain metabolite concentrations consistent with ischemic injury [ 110 ].
RRT is generally advised for critically ill patients when absolute solute/volume criteria are met and medical treatment proves insufficient [ 111 ]. However, RRT initiation should not be delayed in patients with ABI since rapid changes in osmolality could create an osmotic gradient with adverse neurologic consequences. Despite the association between pre-RRT BUN level and subsequent ICP elevation [ 112 ], there is no conclusive evidence on the optimal timing of RRT initiation in patients with ABI. Clinical practice suggests that maintaining pre-dialysis BUN under 30–35 mg/dL with optimized RRT could decrease the risk of increasing ICP during treatment [ 103 ].
RRT modalities
The RRT modalities commonly used in critically ill patients include intermittent hemodialysis (IHD), continuous RRT (CRRT), and prolonged intermittent RRT (PIRRT); (Table 2 ). IHD is typically used three times weekly for 3–4 h per session and allows for rapid solute control and fluid removal. CRRT encompasses various modalities developed specifically for critically ill patients to provide slower solute and fluid removal than IHD and maintain better hemodynamic stability. PIRRT combines the advantages of both, using conventional hemodialysis machines with blood-pump speeds and dialysate flow rates between IHD and CRRT. PIRRT improves hemodynamic stability through slow solute and fluid removal while avoiding the need for 24-h therapy (Table 3 ).
CRRT is less likely to cause DDS and intradialytic hypotension than IHD [ 112 , 115 ] as its slower blood and dialysate/replacement fluid flow rates and smaller dialyzer surface area result in decreased urea clearance from the plasma (Table 3 ). Brain computed tomography studies showed increased brain water content after IHD but not after CRRT [ 116 ]. Moreover, CRRT slower net ultrafiltration rate facilitates hemodynamic stability, prevents intradialytic hypotension, and maintains CPP and brain tissue oxygenation. Although IHD remains an option in patients with mild brain injury and stable conditions, the 2012 KDIGO guidelines recommend CRRT for patients with ABI requiring RRT [ 6 ].
There are several strategies to prevent intradialytic hypotension. Common ones include establishing and adjusting the patient’s dry (target) weight; more frequent and longer RRT sessions to be able to decrease ultrafiltration rates; the avoidance of meals during RRT to mitigate the postprandial drop in blood pressure; the preemptive pharmacological use of midodrine, droxidopa, or sertraline; the use of cool dialysate; sodium profiling; and the use of a high calcium bath [ 117 , 118 , 119 ].
Conclusive evidence comparing the clinical outcomes after CRRT versus PIRRT in patients with ABI is lacking. A study in patients with brain hemorrhage found similar effects on hemodynamics and ICP [ 120 ]. PIRRT is potentially more efficient in resource utilization and offers greater patient care flexibility since it requires a standard IHD device and its administration time is shorter [ 121 ]. While the PIRRT effect is intermediate between IHD and CRRT, it could be an alternative to CRRT in patients who might not necessarily require CRRT or with concerns regarding IHD use in ABI and in centers without CRRT availability [ 68 ].
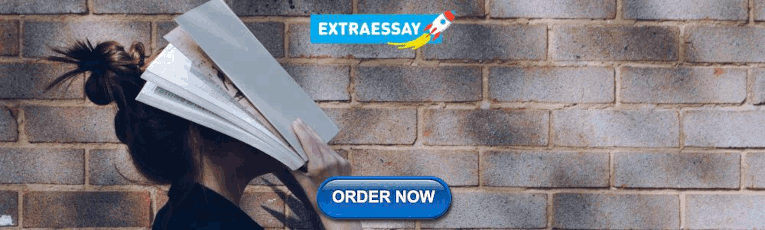
Sodium regulation with RRT
Sodium-based osmotherapy is crucial in managing cerebral edema and preventing ICP increase in patients with ABI. However, the standard sodium concentration in CRRT solutions is 140 mmol/L, lower than the recommended serum sodium concentration to maintain the osmotic pressure between the brain and the plasma (145–155 mmol/L). Sodium concentration in CRRT can be adjusted by adding hypertonic saline (NaCl 23.4%) to the CRRT solution bags or by administering hypertonic saline (NaCl 3%) as CRRT post-filter replacement fluid or as a separate infusion (Table 4 ). In IHD, a 145 mmol/L sodium bath and a separate hypertonic saline infusion could help achieve the desired serum sodium concentration.
Anticoagulation for RRT
Systemic anticoagulation for RRT should be avoided in patients with ABI. While most intermittent IHD sessions can be conducted without anticoagulation, regional citrate anticoagulation (RCA) is recommended for CRRT [ 68 ]. However, some considerations are important. The use of citrate can lead to either metabolic acidosis or alkalosis, depending on the ability of the liver to metabolize citrate. Additionally, due to its calcium-chelating effect, careful calcium replacement is necessary to prevent neurotoxicity stemming from low ionized calcium levels in the patient. RCA should not be used in cases of shock liver or lactic acidosis exceeding 4 mmol/L due to citrate accumulation. Therefore, careful monitoring of acid–base status, calcium, and lactic acid is recommended. If RCA is unavailable or contraindicated, CRRT can be performed without anticoagulation. Although nafamostat could be an alternative anticoagulant [ 123 , 124 , 125 , 126 ], its efficacy in multiple CRRT clinical settings, including ABI, requires further evaluation.
Conclusions
AKI, common in neurocritical patients, is associated with increased morbidity and mortality and has significant implications for managing ABI and its sequelae. Prompt identification of the cause of AKI, with a focus on reversible factors, and the adoption of preventive measures are crucial. The use of RRT in ABI patients is challenging due to potential negative impacts on ICP, CPP, brain oxygenation, and more. CRRT is preferred in ABI cases for gradual solute, electrolyte, and volume adjustments. Unless contraindicated, RCA should be considered for anticoagulation during CRRT. When CRRT is unavailable, intermittent dialysis methods with careful adjustments might be used to minimize complications. Given the magnitude of the problem, future research should focus on better understanding the mechanisms leading to AKI during ABI, and optimizing AKI management in neurocritical care.
Availability of data and materials
Not applicable.
Abbreviations
Acute brain injury
Acute kidney injury
Antihypertensive treatment of acute cerebral hemorrhage II
Blood pressure
Chronic kidney disease
Continuous hyperosmolar therapy for traumatic brain-injured patients
Cerebral perfusion pressure
Continuous renal replacement therapy
- Dialysis disequilibrium syndrome
Erythropoetin in traumatic brain injury
European Society of Intensive Care Medicine
Glomerular filtration rate
- Intracerebral hemorrhage
Intracranial pressure
Intensive care unit
Intermittent hemodialysis
Kidney Disease: Improving Global Outcomes
Mean arterial pressure
Neurocritical Care Society
Prolonged intermittent renal replacement therapy
Regional citrate anticoagulation
- Subarachnoid hemorrhage
Systolic blood pressure
Serum creatinine
- Traumatic brain injury
Urinary output
Griffin BR, Liu KD, Teixeira JP. Critical care nephrology: core curriculum 2020. Am J Kidney Dis. 2020;75(3):435–52.
Article PubMed PubMed Central Google Scholar
Kellum JA, Ronco C, Bellomo R. Conceptual advances and evolving terminology in acute kidney disease. Nat Rev Nephrol. 2021;17(7):493–502.
Article CAS PubMed Google Scholar
James MT, Bhatt M, Pannu N, Tonelli M. Long-term outcomes of acute kidney injury and strategies for improved care. Nat Rev Nephrol. 2020;16(4):193–205.
Article PubMed Google Scholar
Bellomo R, Ronco C, Kellum JA, Mehta RL, Palevsky P, Acute Dialysis Quality Initiative workgroup. Acute renal failure–definition, outcome measures, animal models, fluid therapy and information technology needs: the Second International Consensus Conference of the Acute Dialysis Quality Initiative (ADQI) Group. Crit Care. 2004;8(4):R204-212.
Mehta RL, Kellum JA, Shah SV, Molitoris BA, Ronco C, Warnock DG, Levin A, Acute Kidney Injury Network. Acute Kidney Injury Network: report of an initiative to improve outcomes in acute kidney injury. Crit Care. 2007;11(2):R31.
Kidney Disease: Improving Global Outcomes (KDIGO) Acute Kidney Injury Work Group. KDIGO Clinical Practice Guideline for Acute Kidney Injury. Kidney Int Suppl. 2012;2(1):1–138.
Vanmassenhove J, Steen J, Vansteelandt S, Morzywolek P, Hoste E, Decruyenaere J, Benoit D, Van Biesen W. The importance of the urinary output criterion for the detection and prognostic meaning of AKI. Sci Rep. 2021;11(1):11089.
Article CAS PubMed PubMed Central Google Scholar
Hoste EA, Bagshaw SM, Bellomo R, Cely CM, Colman R, Cruz DN, Edipidis K, Forni LG, Gomersall CD, Govil D, et al. Epidemiology of acute kidney injury in critically ill patients: the multinational AKI-EPI study. Intensive Care Med. 2015;41(8):1411–23.
Ramirez-Guerrero G, Lucero C, Villagran-Cortes F, Hauway E, Torres-Cifuentes V, Baghetti-Hernandez R, Vera-Calzaretta A, Ronco C, Garay O. Acute kidney injury in neurocritical patients: a retrospective cohort study. Int Urol Nephrol. 2023;55(7):1875–83.
Robba C, Banzato E, Rebora P, Iaquaniello C, Huang CY, Wiegers EJA, Meyfroidt G, Citerio G, Collaborative European NeuroTrauma Effectiveness Research in Traumatic Brain Injury (TBI) ICU Participants and Investigators. Acute kidney injury in traumatic brain injury patients: results from the collaborative European neurotrauma effectiveness research in traumatic brain injury study. Crit Care Med. 2021;49(1):112–26.
Skrifvars MB, Bailey M, Moore E, Martensson J, French C, Presneill J, Nichol A, Little L, Duranteau J, Huet O, et al. A post hoc analysis of osmotherapy use in the erythropoietin in traumatic brain injury study-associations with acute kidney injury and mortality. Crit Care Med. 2021;49(4):e394–403.
Wang R, Zhang J, Xu J, He M, Xu J. Incidence and burden of acute kidney injury among traumatic brain-injury patients. Risk Manag Healthc Policy. 2021;14:4571–80.
Liu Z, Wang R, He M, Kang Y. Hypomagnesemia is associated with the acute kidney injury in traumatic brain injury patients: a pilot study. Brain Sci. 2023;13(4):593.
Huet O, Chapalain X, Vermeersch V, Moyer JD, Lasocki S, Cohen B, Dahyot-Fizelier C, Chalard K, Seguin P, Hourmant Y, et al. Impact of continuous hypertonic (NaCl 20%) saline solution on renal outcomes after traumatic brain injury (TBI): a post hoc analysis of the COBI trial. Crit Care. 2023;27(1):42.
Huang ZY, Liu Y, Huang HF, Huang SH, Wang JX, Tian JF, Zeng WX, Lv RG, Jiang S, Gao JL, et al. Acute kidney injury in traumatic brain injury intensive care unit patients. World J Clin Cases. 2022;10(9):2751–63.
Wang D, Guo Y, Zhang Y, Li Z, Li A, Luo Y. Epidemiology of acute kidney injury in patients with stroke: a retrospective analysis from the neurology ICU. Intern Emerg Med. 2018;13(1):17–25.
Gwak DS, Chung I, Kim BK, Lee S, Jeong HG, Kim YS, Chae H, Park CY, Han MK. High chloride burden and clinical outcomes in critically ill patients with large hemispheric infarction. Front Neurol. 2021;12:604686.
Sadan O, Singbartl K, Kandiah PA, Martin KS, Samuels OB. Hyperchloremia is associated with acute kidney injury in patients with subarachnoid hemorrhage. Crit Care Med. 2017;45(8):1382–8.
Sadan O, Singbartl K, Kraft J, Plancher JM, Greven ACM, Kandiah P, Pimentel C, Hall CL, Papangelou A, Asbury WH, et al. Low-chloride- versus high-chloride-containing hypertonic solution for the treatment of subarachnoid hemorrhage-related complications: the ACETatE (A low ChloriE hyperTonic solution for brain Edema) randomized trial. J Intensive Care. 2020;8:32.
Riha HM, Erdman MJ, Vandigo JE, Kimmons LA, Goyal N, Davidson KE, Pandhi A, Jones GM. Impact of moderate hyperchloremia on clinical outcomes in intracerebral hemorrhage patients treated with continuous infusion hypertonic saline: a pilot study. Crit Care Med. 2017;45(9):e947–53.
Burgess LG, Goyal N, Jones GM, Khorchid Y, Kerro A, Chapple K, Tsivgoulis G, Alexandrov AV, Chang JJ. Evaluation of acute kidney injury and mortality after intensive blood pressure control in patients with intracerebral hemorrhage. J Am Heart Assoc. 2018;7(8):e008439.
Tujjar O, Belloni I, Hougardy JM, Scolletta S, Vincent JL, Creteur J, Taccone FS. Acute kidney injury after subarachnoid hemorrhage. J Neurosurg Anesthesiol. 2017;29(2):140–9.
Kumar AB, Shi Y, Shotwell MS, Richards J, Ehrenfeld JM. Hypernatremia is a significant risk factor for acute kidney injury after subarachnoid hemorrhage: a retrospective analysis. Neurocrit Care. 2015;22(2):184–91.
Luu D, Komisarow J, Mills BM, Vavilala MS, Laskowitz DT, Mathew J, James ML, Hernandez A, Sampson J, Fuller M, et al. Association of severe acute kidney injury with mortality and healthcare utilization following isolated traumatic brain injury. Neurocrit Care. 2021;35(2):434–40.
Zacharia BE, Ducruet AF, Hickman ZL, Grobelny BT, Fernandez L, Schmidt JM, Narula R, Ko LN, Cohen ME, Mayer SA, et al. Renal dysfunction as an independent predictor of outcome after aneurysmal subarachnoid hemorrhage: a single-center cohort study. Stroke. 2009;40(7):2375–81.
Buttner S, Stadler A, Mayer C, Patyna S, Betz C, Senft C, Geiger H, Jung O, Finkelmeier F. Incidence, risk factors, and outcome of acute kidney injury in neurocritical care. J Intensive Care Med. 2020;35(4):338–46.
Nadkarni GN, Patel AA, Konstantinidis I, Mahajan A, Agarwal SK, Kamat S, Annapureddy N, Benjo A, Thakar CV. Dialysis requiring acute kidney injury in acute cerebrovascular accident hospitalizations. Stroke. 2015;46(11):3226–31.
Murray AM. Cognitive impairment in the aging dialysis and chronic kidney disease populations: an occult burden. Adv Chronic Kidney Dis. 2008;15(2):123–32.
Lee M, Saver JL, Chang KH, Liao HW, Chang SC, Ovbiagele B. Low glomerular filtration rate and risk of stroke: meta-analysis. BMJ. 2010;341:c4249.
Kumai Y, Kamouchi M, Hata J, Ago T, Kitayama J, Nakane H, Sugimori H, Kitazono T, FSR Investigators. FSR investigators proteinuria and clinical outcomes after ischemic stroke. Neurology. 2012;78(24):1909–15.
Beuscher VD, Sprugel MI, Gerner ST, Sembill JA, Madzar D, Reindl C, Lucking H, Lang S, Hoelter P, Kuramatsu JB, et al. Chronic kidney disease and clinical outcomes in patients with intracerebral hemorrhage. J Stroke Cerebrovasc Dis. 2020;29(8):104802.
Ng L, Wang J, Altaweel L, Athar MK. Neurologic aspects of cardiac emergencies. Crit Care Clin. 2014;30(3):557–84.
Prunet B, Basely M, D’Aranda E, Cambefort P, Pons F, Cimarelli S, Dagain A, Desse N, Veyrieres JB, Jego C, et al. Impairment of cardiac metabolism and sympathetic innervation after aneurysmal subarachnoid hemorrhage: a nuclear medicine imaging study. Crit Care. 2014;18(3):R131.
Nguyen H, Zaroff JG. Neurogenic stunned myocardium. Curr Neurol Neurosci Rep. 2009;9(6):486–91.
Kerro A, Woods T, Chang JJ. Neurogenic stunned myocardium in subarachnoid hemorrhage. J Crit Care. 2017;38:27–34.
Messerer DAC, Halbgebauer R, Nilsson B, Pavenstadt H, Radermacher P, Huber-Lang M. Immunopathophysiology of trauma-related acute kidney injury. Nat Rev Nephrol. 2021;17(2):91–111.
Cook AM, Morgan Jones G, Hawryluk GWJ, Mailloux P, McLaughlin D, Papangelou A, Samuel S, Tokumaru S, Venkatasubramanian C, Zacko C, et al. Guidelines for the acute treatment of cerebral edema in neurocritical care patients. Neurocrit Care. 2020;32(3):647–66.
Lin SY, Tang SC, Tsai LK, Yeh SJ, Shen LJ, Wu FL, Jeng JS. Incidence and risk factors for acute kidney injury following mannitol infusion in patients with acute stroke: a retrospective cohort study. Medicine (Baltimore). 2015;94(47):e2032.
Kim MY, Park JH, Kang NR, Jang HR, Lee JE, Huh W, Kim YG, Kim DJ, Hong SC, Kim JS, et al. Increased risk of acute kidney injury associated with higher infusion rate of mannitol in patients with intracranial hemorrhage. J Neurosurg. 2014;120(6):1340–8.
Fang L, You H, Chen B, Xu Z, Gao L, Liu J, Xie Q, Zhou Y, Gu Y, Lin S, et al. Mannitol is an independent risk factor of acute kidney injury after cerebral trauma: a case–control study. Ren Fail. 2010;32(6):673–9.
Gondim Fde A, Aiyagari V, Shackleford A, Diringer MN. Osmolality not predictive of mannitol-induced acute renal insufficiency. J Neurosurg. 2005;103(3):444–7.
Fink ME. Osmotherapy for intracranial hypertension: mannitol versus hypertonic saline. Continuum (Minneap Minn). 2012;18(3):640–54.
PubMed Google Scholar
Erdman MJ, Riha H, Bode L, Chang JJ, Jones GM. Predictors of acute kidney injury in neurocritical care patients receiving continuous hypertonic saline. Neurohospitalist. 2017;7(1):9–14.
Sigmon J, May CC, Bryant A, Humanez J, Singh V. Assessment of acute kidney injury in neurologically injured patients receiving hypertonic sodium chloride: Does chloride load matter? Ann Pharmacother. 2020;54(6):541–6.
Semler MW, Kellum JA. Balanced crystalloid solutions. Am J Respir Crit Care Med. 2019;199(8):952–60.
Swenson ER. Balanced crystalloid versus saline solution in critically ill patients: Is chloride the villain? Am J Respir Crit Care Med. 2019;200(3):398.
Hansen PB, Jensen BL, Skott O. Chloride regulates afferent arteriolar contraction in response to depolarization. Hypertension. 1998;32(6):1066–70.
Wilcox CS. Regulation of renal blood flow by plasma chloride. J Clin Invest. 1983;71(3):726–35.
Chowdhury AH, Cox EF, Francis ST, Lobo DN. A randomized, controlled, double-blind crossover study on the effects of 2-L infusions of 0.9% saline and plasma-lyte(R) 148 on renal blood flow velocity and renal cortical tissue perfusion in healthy volunteers. Ann Surg. 2012;256(1):18–24.
Davenport MS, Perazella MA, Yee J, Dillman JR, Fine D, McDonald RJ, Rodby RA, Wang CL, Weinreb JC. Use of intravenous iodinated contrast media in patients with kidney disease: consensus statements from the American College of Radiology and the National Kidney Foundation. Radiology. 2020;294(3):660–8.
McDonald JS, McDonald RJ, Williamson EE, Kallmes DF, Kashani K. Post-contrast acute kidney injury in intensive care unit patients: a propensity score-adjusted study. Intensive Care Med. 2017;43(6):774–84.
Schonenberger E, Martus P, Bosserdt M, Zimmermann E, Tauber R, Laule M, Dewey M. Kidney injury after intravenous versus intra-arterial contrast agent in patients suspected of having coronary artery disease: a randomized trial. Radiology. 2019;292(3):664–72.
Corral L, Javierre CF, Ventura JL, Marcos P, Herrero JI, Manez R. Impact of non-neurological complications in severe traumatic brain injury outcome. Crit Care. 2012;16(2):R44.
Barea-Mendoza JA, Chico-Fernandez M, Quintana-Diaz M, Servia-Goixart L, Fernandez-Cuervo A, Bringas-Bollada M, Ballesteros-Sanz MA, Garcia-Saez I, Perez-Barcena J, Llompart-Pou JA, et al. Traumatic brain injury and acute kidney injury-outcomes and associated risk factors. J Clin Med. 2022;11(23):7216.
Adachi N, Lei B, Deshpande G, Seyfried FJ, Shimizu I, Nagaro T, Arai T. Uraemia suppresses central dopaminergic metabolism and impairs motor activity in rats. Intensive Care Med. 2001;27(10):1655–60.
Jeppsson B, Freund HR, Gimmon Z, James JH, von Meyenfeldt MF, Fischer JE. Blood-brain barrier derangement in uremic encephalopathy. Surgery. 1982;92(1):30–5.
CAS PubMed Google Scholar
Trachtman H, Futterweit S, Tonidandel W, Gullans SR. The role of organic osmolytes in the cerebral cell volume regulatory response to acute and chronic renal failure. J Am Soc Nephrol. 1993;3(12):1913–9.
Silver SM. Cerebral edema after rapid dialysis is not caused by an increase in brain organic osmolytes. J Am Soc Nephrol. 1995;6(6):1600–6.
Liu M, Liang Y, Chigurupati S, Lathia JD, Pletnikov M, Sun Z, Crow M, Ross CA, Mattson MP, Rabb H. Acute kidney injury leads to inflammation and functional changes in the brain. J Am Soc Nephrol. 2008;19(7):1360–70.
Palkovits M, Sebekova K, Gallatz K, Boor P, Sebekova K Jr, Klassen A, Bahner U, Heidland A. Neuronal activation in the CNS during different forms of acute renal failure in rats. Neuroscience. 2009;159(2):862–82.
Salama M, Farrag SM, abulasrar S, Amin MM, Ali AA, Sheashaa H, Sobh M, Arias-Carrion O. Up-regulation of TLR-4 in the brain after ischemic kidney-induced encephalopathy in the rat. CNS Neurol Disord Drug Targets. 2013;12(5):583–586.
Chou AH, Lee CM, Chen CY, Liou JT, Liu FC, Chen YL, Day YJ. Hippocampal transcriptional dysregulation after renal ischemia and reperfusion. Brain Res. 2014;1582:197–210.
Andres-Hernando A, Dursun B, Altmann C, Ahuja N, He Z, Bhargava R, Edelstein CE, Jani A, Hoke TS, Klein C, et al. Cytokine production increases and cytokine clearance decreases in mice with bilateral nephrectomy. Nephrol Dial Transplant. 2012;27(12):4339–47.
Chen J, Hartono JR, John R, Bennett M, Zhou XJ, Wang Y, Wu Q, Winterberg PD, Nagami GT, Lu CY. Early interleukin 6 production by leukocytes during ischemic acute kidney injury is regulated by TLR4. Kidney Int. 2011;80(5):504–15.
Lu R, Kiernan MC, Murray A, Rosner MH, Ronco C. Kidney-brain crosstalk in the acute and chronic setting. Nat Rev Nephrol. 2015;11(12):707–19.
Nongnuch A, Panorchan K, Davenport A. Brain-kidney crosstalk. Crit Care. 2014;18(3):225.
Ramirez-Guerrero G, Baghetti-Hernandez R, Ronco C. Acute kidney injury at the neurocritical care unit. Neurocrit Care. 2021;36:640–9.
Khwaja A. KDIGO clinical practice guidelines for acute kidney injury. Nephron Clin Pract. 2012;120(4):c179-184.
Bouma GJ, Muizelaar JP, Bandoh K, Marmarou A. Blood pressure and intracranial pressure-volume dynamics in severe head injury: relationship with cerebral blood flow. J Neurosurg. 1992;77(1):15–9.
Asfar P, Meziani F, Hamel JF, Grelon F, Megarbane B, Anguel N, Mira JP, Dequin PF, Gergaud S, Weiss N, et al. High versus low blood-pressure target in patients with septic shock. New Engl J Med. 2014;370(17):1583–93.
Chesnut R, Aguilera S, Buki A, Bulger E, Citerio G, Cooper DJ, Arrastia RD, Diringer M, Figaji A, Gao G, et al. A management algorithm for adult patients with both brain oxygen and intracranial pressure monitoring: the Seattle International Severe Traumatic Brain Injury Consensus Conference (SIBICC). Intensive Care Med. 2020;46(5):919–29.
Carney N, Totten AM, O’Reilly C, Ullman JS, Hawryluk GW, Bell MJ, Bratton SL, Chesnut R, Harris OA, Kissoon N, et al. Guidelines for the management of severe traumatic brain injury, Fourth edition. Neurosurgery. 2017;80(1):6–15.
Evans L, Rhodes A, Alhazzani W, Antonelli M, Coopersmith CM, French C, Machado FR, McIntyre L, Ostermann M, Prescott HC, et al. Surviving sepsis campaign: international guidelines for management of sepsis and septic shock 2021. Intensive Care Med. 2021;47(11):1181–247.
Huijben JA, Volovici V, Cnossen MC, Haitsma IK, Stocchetti N, Maas AIR, Menon DK, Ercole A, Citerio G, Nelson D, et al. Variation in general supportive and preventive intensive care management of traumatic brain injury: a survey in 66 neurotrauma centers participating in the Collaborative European NeuroTrauma Effectiveness Research in Traumatic Brain Injury (CENTER-TBI) study. Crit Care. 2018;22(1):90.
Steiner LA, Siegemund M. Vasoactive agents to improve brain perfusion: pathophysiology and clinical utilization. Curr Opin Crit Care. 2019;25(2):110–6.
Bhatraju PK, Zelnick LR, Herting J, Katz R, Mikacenic C, Kosamo S, Morrell ED, Robinson-Cohen C, Calfee CS, Christie JD, et al. Identification of acute kidney injury subphenotypes with differing molecular signatures and responses to vasopressin therapy. Am J Respir Crit Care Ced. 2019;199(7):863–72.
Article CAS Google Scholar
Greenberg SM, Ziai WC, Cordonnier C, Dowlatshahi D, Francis B, Goldstein JN, Hemphill JC 3rd, Johnson R, Keigher KM, Mack WJ, et al. 2022 Guideline for the management of patients with spontaneous intracerebral hemorrhage: a guideline from the American Heart Association/American Stroke Association. Stroke. 2022;53(7):e282–361.
Ravera M, Re M, Deferrari L, Vettoretti S, Deferrari G. Importance of blood pressure control in chronic kidney disease. J Am Soc Nephrol. 2006;17(4 Suppl 2):S98-103.
Qureshi AI, Huang W, Lobanova I, Barsan WG, Hanley DF, Hsu CY, Lin CL, Silbergleit R, Steiner T, Suarez JI, et al. Outcomes of intensive systolic blood pressure reduction in patients with intracerebral hemorrhage and excessively high initial systolic blood pressure: post hoc analysis of a randomized clinical trial. JAMA Neurol. 2020;77(11):1355–65.
Qureshi AI, Huang W, Lobanova I, Hanley DF, Hsu CY, Malhotra K, Steiner T, Suarez JI, Toyoda K, Yamamoto H, et al. Systolic blood pressure reduction and acute kidney injury in intracerebral hemorrhage. Stroke. 2020;51(10):3030–8.
Clifton GL, Miller ER, Choi SC, Levin HS. Fluid thresholds and outcome from severe brain injury. Crit Care Med. 2002;30(4):739–45.
Clifton GL, Valadka A, Zygun D, Coffey CS, Drever P, Fourwinds S, Janis LS, Wilde E, Taylor P, Harshman K, et al. Very early hypothermia induction in patients with severe brain injury (the National Acute Brain Injury Study: Hypothermia II): a randomised trial. Lancet Neurol. 2011;10(2):131–9.
Wiegers EJA, Lingsma HF, Huijben JA, Cooper DJ, Citerio G, Frisvold S, Helbok R, Maas AIR, Menon DK, Moore EM, et al. Fluid balance and outcome in critically ill patients with traumatic brain injury (CENTER-TBI and OzENTER-TBI): a prospective, multicentre, comparative effectiveness study. Lancet Neurol. 2021;20(8):627–38.
Husain-Syed F, Grone HJ, Assmus B, Bauer P, Gall H, Seeger W, Ghofrani A, Ronco C, Birk HW. Congestive nephropathy: a neglected entity? Proposal for diagnostic criteria and future perspectives. ESC Heart Fail. 2021;8(1):183–203.
Oddo M, Poole D, Helbok R, Meyfroidt G, Stocchetti N, Bouzat P, Cecconi M, Geeraerts T, Martin-Loeches I, Quintard H, et al. Fluid therapy in neurointensive care patients: ESICM consensus and clinical practice recommendations. Intensive Care Med. 2018;44(4):449–63.
Romero-Gonzalez G, Manrique J, Slon-Roblero MF, Husain-Syed F, De la Espriella R, Ferrari F, Bover J, Ortiz A, Ronco C. PoCUS in nephrology: a new tool to improve our diagnostic skills. Clin Kidney J. 2023;16(2):218–29.
Hammond NE, Zampieri FG, Di Tanna GL, Garside T, Adigbli D, Cavalcanti AB, Machado FR, Micallef S, Myburgh J, Ramanan M, et al. Balanced crystalloids versus saline in critically ill adults—a systematic review with meta-analysis. NEJM Evid. 2022. https://doi.org/10.1056/EVIDoa2100010 .
Article Google Scholar
Lehmann L, Bendel S, Uehlinger DE, Takala J, Schafer M, Reinert M, Jakob SM. Randomized, double-blind trial of the effect of fluid composition on electrolyte, acid-base, and fluid homeostasis in patients early after subarachnoid hemorrhage. Neurocrit Care. 2013;18(1):5–12.
Roquilly A, Loutrel O, Cinotti R, Rosenczweig E, Flet L, Mahe PJ, Dumont R, Marie Chupin A, Peneau C, Lejus C, et al. Balanced versus chloride-rich solutions for fluid resuscitation in brain-injured patients: a randomised double-blind pilot study. Crit Care. 2013;17(2):R77.
Reinhart K, Perner A, Sprung CL, Jaeschke R, Schortgen F, Johan Groeneveld AB, Beale R, Hartog CS, European Society of Intensive Care Medicine. Consensus statement of the ESICM task force on colloid volume therapy in critically ill patients. Intensive Care Med. 2012;38(3):368–83.
Maguigan KL, Dennis BM, Hamblin SE, Guillamondegui OD. Method of hypertonic saline administration: effects on osmolality in traumatic brain injury patients. J Clin Neurosci. 2017;39:147–50.
Chau K, Yong J, Ismail K, Griffith N, Liu M, Makris A. Levetiracetam-induced severe acute granulomatous interstitial nephritis. Clin Kidney J. 2012;5(3):234–6.
Rossert J. Drug-induced acute interstitial nephritis. Kidney Int. 2001;60(2):804–17.
Hefny F, Stuart A, Kung JY, Mahmoud SH. Prevalence and risk factors of augmented renal clearance: a systematic review and meta-analysis. Pharmaceutics. 2022;14(2):445.
Udy AA, Jarrett P, Lassig-Smith M, Stuart J, Starr T, Dunlop R, Deans R, Roberts JA, Senthuran S, Boots R, et al. Augmented renal clearance in traumatic brain injury: a single-center observational study of atrial natriuretic peptide, cardiac output, and creatinine clearance. J Neurotrauma. 2017;34(1):137–44.
Chen Y, Liu L, Zhu M. Effect of augmented renal clearance on the therapeutic drug monitoring of vancomycin in patients after neurosurgery. J Int Med Res. 2020;48(10):300060520949076.
Ong CLJ, Goh PSJ, Teo MM, Lim TP, Goh KKK, Ang XY, Lim LJK, Jamaludin NHB, Ang BT, Kwa LHA. Pharmacokinetics of levetiracetam in neurosurgical ICU patients. J Crit Care. 2021;64:255–61.
Ostermann M, Zarbock A, Goldstein S, Kashani K, Macedo E, Murugan R, Bell M, Forni L, Guzzi L, Joannidis M, et al. Recommendations on acute kidney injury biomarkers from the acute disease quality initiative consensus conference: a consensus statement. JAMA Netw Open. 2020;3(10):e2019209.
Li N, Zhao WG, Xu FL, Zhang WF, Gu WT. Neutrophil gelatinase-associated lipocalin as an early marker of acute kidney injury in patients with traumatic brain injury. J Nephrol. 2013;26(6):1083–8.
Shimoyama T, Sato T, Sakamoto Y, Nagai K, Aoki J, Suda S, Nishiyama Y, Kimura K. Urinary biomarkers of kidney tubule injury, risk of acute kidney injury, and mortality in patients with acute ischaemic stroke treated at a stroke care unit. Eur J Neurol. 2020;27(12):2463–72.
Wang S, Xie L, Xu J, Hu Y, Wu Y, Lin Z, Pan S. Predictive value of serum creatinine/cystatin C in neurocritically ill patients. Brain Behav. 2019;9(12):e01462.
Jiang F, Su L, Xiang H, Zhang X, Xu D, Zhang Z, Peng Z. Incidence, risk factors, and biomarkers predicting ischemic or hemorrhagic stroke associated acute kidney injury and outcome: a retrospective study in a general intensive care unit. Blood Purif. 2019;47(4):317–26.
Davenport A. Renal replacement therapy in the patient with acute brain injury. Am J Kidney Dis. 2001;37(3):457–66.
Trinh-Trang-Tan MM, Cartron JP, Bankir L. Molecular basis for the dialysis disequilibrium syndrome: altered aquaporin and urea transporter expression in the brain. Nephrol Dial Transplant. 2005;20(9):1984–8.
Shapiro JI, Whalen M, Kucera R, Kindig N, Filley G, Chan L. Brain pH responses to sodium bicarbonate and Carbicarb during systemic acidosis. Am J Physiol. 1989;256(5 Pt 2):H1316-1321.
Workgroup KD. K/DOQI clinical practice guidelines for cardiovascular disease in dialysis patients. Am J Kidney Dis. 2005;45(4 Suppl 3):S1-153.
Google Scholar
Daugirdas JT. Pathophysiology of dialysis hypotension: an update. Am J Kidney Dis. 2001;38(4 Suppl 4):S11-17.
Ko SB, Choi HA, Gilmore E, Schmidt JM, Claassen J, Lee K, Mayer SA, Badjatia N. Pearls & Oysters: the effects of renal replacement therapy on cerebral autoregulation. Neurology. 2012;78(6):e36-38.
Ross EA, Tashkin D, Chenoweth D, Webber MM, Nissenson AR. Pulmonary leukosequestration without hypoxemia during hemodialysis. Int J Artif Organs. 1987;10(6):367–74.
Anazodo UC, Wong DY, Theberge J, Dacey M, Gomes J, Penny JD, Van Ginkel M, Poirier SE, McIntyre CW. Hemodialysis-Related acute brain injury demonstrated by application of intradialytic magnetic resonance imaging and spectroscopy. J Am Soc Nephrol. 2023;34(6):1090–104.
STARRT-AKI Investigators; Canadian Critical Care Trials Group; Australian and New Zealand Intensive Care Society Clinical Trials Group; United Kingdom Critical Care Research Group; Canadian Nephrology Trials Network; Irish Critical Care Trials Group; Bagshaw SM, Wald R, Adhikari NKJ, et al: Timing of initiation of renal-replacement therapy in acute kidney injury. N Engl J Med. 2020;383(3):240–51.
Lund A, Damholt MB, Wiis J, Kelsen J, Strange DG, Moller K. Intracranial pressure during hemodialysis in patients with acute brain injury. Acta Anaesthesiol Scand. 2019;63(4):493–9.
Beaubien-Souligny W, Trott T, Neyra JA. How to determine fluid management goals during continuous kidney replacement therapy in patients with AKI: focus on POCUS. Kidney360. 2022;3(10):1795–806.
Tsujimoto Y, Tsujimoto H, Nakata Y, Kataoka Y, Kimachi M, Shimizu S, Ikenoue T, Fukuma S, Yamamoto Y, Fukuhara S. Dialysate temperature reduction for intradialytic hypotension for people with chronic kidney disease requiring haemodialysis. Cochrane Database Syst Rev. 2019;7(7):CD012598.
Schneider AG, Bellomo R, Bagshaw SM, Glassford NJ, Lo S, Jun M, Cass A, Gallagher M. Choice of renal replacement therapy modality and dialysis dependence after acute kidney injury: a systematic review and meta-analysis. Intensive Care Med. 2013;39(6):987–97.
Ronco C, Bellomo R, Brendolan A, Pinna V, La Greca G. Brain density changes during renal replacement in critically ill patients with acute renal failure. Continuous hemofiltration versus intermittent hemodialysis. J Nephrol. 1999;12(3):173–8.
Douvris A, Malhi G, Hiremath S, McIntyre L, Silver SA, Bagshaw SM, Wald R, Ronco C, Sikora L, Weber C, et al. Interventions to prevent hemodynamic instability during renal replacement therapy in critically ill patients: a systematic review. Crit Care. 2018;22(1):41.
Flythe JE, Chang TI, Gallagher MP, Lindley E, Madero M, Sarafidis PA, Unruh ML, Wang AY, Weiner DE, Cheung M, et al. Blood pressure and volume management in dialysis: conclusions from a kidney disease: improving global outcomes (KDIGO) controversies conference. Kidney Int. 2020;97(5):861–76.
Chou JA, Kalantar-Zadeh K, Mathew AT. A brief review of intradialytic hypotension with a focus on survival. Sem Dial. 2017;30(6):473–80.
Wu VC, Huang TM, Shiao CC, Lai CF, Tsai PR, Wang WJ, Huang HY, Wang KC, Ko WJ, Wu KD, et al. The hemodynamic effects during sustained low-efficiency dialysis versus continuous veno-venous hemofiltration for uremic patients with brain hemorrhage: a crossover study. J Neurosurg. 2013;119(5):1288–95.
Schwenger V, Weigand MA, Hoffmann O, Dikow R, Kihm LP, Seckinger J, Miftari N, Schaier M, Hofer S, Haar C, et al. Sustained low efficiency dialysis using a single-pass batch system in acute kidney injury—a randomized interventional trial: the REnal Replacement Therapy Study in Intensive Care Unit PatiEnts. Crit Care. 2012;16(4):R140.
Yessayan LT, Szamosfalvi B, Rosner MH. Management of dysnatremias with continuous renal replacement therapy. Sem Dial. 2021;34(6):472–9.
Choi JY, Kang YJ, Jang HM, Jung HY, Cho JH, Park SH, Kim YL, Kim CD. Nafamostat mesilate as an anticoagulant during continuous renal replacement therapy in patients with high bleeding risk: a randomized clinical trial. Medicine (Baltimore). 2015;94(52):e2392.
Chen T, Wang J, Li C, Zhang W, Zhang L, An L, Pang T, Shi X, Liao H. Nafamostat mesilate attenuates neuronal damage in a rat model of transient focal cerebral ischemia through thrombin inhibition. Sci Rep. 2014;4:5531.
Matsubara H, Imai T, Tsuji S, Oka N, Egashira Y, Enomoto Y, Nakayama N, Nakamura S, Shimazawa M, Iwama T, et al. Nafamostat protects against early brain injury after subarachnoid hemorrhage in mice. J Pharmacol Sci. 2022;148(1):65–72.
Yang JW, Han BG, Kim BR, Lee YH, Kim YS, Yu JM, Choi SO. Superior outcome of nafamostat mesilate as an anticoagulant in patients undergoing maintenance hemodialysis with intracerebral hemorrhage. Ren Fail. 2009;31(8):668–75.
Download references
Acknowledgements
This work did not receive any specific grant from funding agencies in the public, commercial, or not-for-profit sectors.
Author information
Faeq Husain-Syed and Tomonori Takeuchi are joint first authors.
Authors and Affiliations
Division of Nephrology, University of Virginia School of Medicine, 1300 Jefferson Park Avenue, Charlottesville, VA, 22908, USA
Faeq Husain-Syed & Mitchell H. Rosner
Department of Internal Medicine II, University Hospital Giessen and Marburg, Justus-Liebig-University Giessen, Klinikstrasse 33, 35392, Giessen, Germany
Faeq Husain-Syed
Division of Nephrology, University of Alabama at Birmingham, 1720 2nd Avenue South, Birmingham, AL, 35294, USA
Tomonori Takeuchi, Javier A. Neyra & Ashita J. Tolwani
Department of Health Policy and Informatics, Tokyo Medical and Dental University, 1-5-45 Yushima, Bunkyo Ku, Tokyo, 113-8510, Japan
Tomonori Takeuchi
Critical Care Unit, Carlos Van Buren Hospital, San Ignacio 725, Valparaíso, Chile
Gonzalo Ramírez-Guerrero
Dialysis and Renal Transplant Unit, Carlos Van Buren Hospital, San Ignacio 725, Valparaíso, Chile
Department of Medicine, Universidad de Valparaíso, Hontaneda 2653, Valparaíso, Chile
Department of Medicine (DIMED), Università di Padova, Via Giustiniani, 2, 35128, Padua, Italy
Claudio Ronco
International Renal Research Institute of Vicenza, Department of Nephrology, Dialysis and Transplantation, San Bortolo Hospital, Via Rodolfi, 37, 36100, Vicenza, Italy
You can also search for this author in PubMed Google Scholar
Contributions
FH-S, TT, JAN, GR-G, and AT prepared all manuscript drafts and were involved in reviewing and editing, including the tables and figures. MHR and CR conceived the concept underlying the manuscript and were involved in editing of the manuscript, including the tables and figures. AT is the paper's senior author. All authors approved the final version of the manuscript.
Corresponding author
Correspondence to Ashita J. Tolwani .
Ethics declarations
Ethics approval and consent to participate, consent for publication, competing interests.
JAN reports consultancy agreements with Baxter Healthcare, Outset Medical, Vifor Pharma, and Leadiant Biosciences, all unrelated to this work. AT reports consultancy for Baxter, receiving honoraria from UptoDate, having a patent on 0.5% trisodium citrate solution for CRRT anti- coagulation, the license for which has been bought by Baxter, and serving on a speakers bureau for Baxter, all unrelated to this work. MHR has received consultant fees from Baxter Healthcare, serves as on the Data Safety Monitoring Boards of clinical trials sponsored by Reata, Travere and Astra Zeneca, all unrelated to this work. CR has received funding for lectures been consultant or advisory board member for Asahi, Astute, B. Braun, Baxter, bioMérieux, Bioporto, CytoSorbents, Estor, Fresenius Medical Care, General Electric (GE), Jafron, Medtronic, Toray. FH-S, TT, and GR-G declare no competing interests.
Additional information
Publisher's note.
Springer Nature remains neutral with regard to jurisdictional claims in published maps and institutional affiliations.
Supplementary Information
Additional file 1: appendix table 1..
Common causes of AKI in the ICU setting.
Additional file 2.
Appendix Literature Search Strategy.
Additional file 3: Appendix Table 2.
AKI and RRT rates and associated outcomes in neurocritically ill patients.
Additional file 4: Appendix Table 3.
Current and proposed definition and staging of AKI.
Additional file 5: Appendix Table 4.
Characteristics of novel AKI biomarkers.
Rights and permissions
Open Access This article is licensed under a Creative Commons Attribution 4.0 International License, which permits use, sharing, adaptation, distribution and reproduction in any medium or format, as long as you give appropriate credit to the original author(s) and the source, provide a link to the Creative Commons licence, and indicate if changes were made. The images or other third party material in this article are included in the article's Creative Commons licence, unless indicated otherwise in a credit line to the material. If material is not included in the article's Creative Commons licence and your intended use is not permitted by statutory regulation or exceeds the permitted use, you will need to obtain permission directly from the copyright holder. To view a copy of this licence, visit http://creativecommons.org/licenses/by/4.0/ . The Creative Commons Public Domain Dedication waiver ( http://creativecommons.org/publicdomain/zero/1.0/ ) applies to the data made available in this article, unless otherwise stated in a credit line to the data.
Reprints and permissions
About this article
Cite this article.
Husain-Syed, F., Takeuchi, T., Neyra, J.A. et al. Acute kidney injury in neurocritical care. Crit Care 27 , 341 (2023). https://doi.org/10.1186/s13054-023-04632-1
Download citation
Received : 18 April 2023
Accepted : 30 August 2023
Published : 03 September 2023
DOI : https://doi.org/10.1186/s13054-023-04632-1
Share this article
Anyone you share the following link with will be able to read this content:
Sorry, a shareable link is not currently available for this article.
Provided by the Springer Nature SharedIt content-sharing initiative
Critical Care
ISSN: 1364-8535
- Submission enquiries: [email protected]
ORIGINAL RESEARCH article
Parental recovered acute kidney injury causes prenatal renal dysfunction and fetal growth restriction with sexually dimorphic implications for adult offspring.
- 1 Department of Anesthesiology and Perioperative Medicine, Oregon Health and Science University, Portland, OR, United States
- 2 Division of Nephrology, Oregon Health and Science University, Portland, OR, United States
- 3 Operative Care Division, Portland Veterans Administration Medical Center, Portland, OR, United States
- 4 Department of Pathology, Oregon Health and Science University, Portland, OR, United States
- 5 Division of Nephrology and Hypertension, Department of Medicine, Keck School Medicine of University of Southern California, Los Angeles, CA, United States
Introduction: Acute kidney injury (AKI) is rapidly increasing in global incidence and a healthcare burden. Prior maternal AKI diagnosis correlates with later pregnancy complications. As pregnancy influences developmental programming, we hypothesized that recovered parental AKI results in poor pregnancy outcomes, impaired fetal growth, and adult offspring disease.
Methods: Using a well-characterized model of rhabdomyolysis-induced acute kidney injury (RIAKI), a form of AKI commonly observed in young people, we confirmed functional renal recovery by assessing glomerular filtration rate (GFR) 2 weeks following RIAKI. We bred sham and recovered RIAKI sires and dams in timed, matched matings for gestational day (GD) 16.5 and offspring (birth–12 weeks, 6 months) study.
Results: Despite a normal GFR pre-pregnancy, recovered RIAKI dams at GD16.5 had impaired renal function, resulting in reduced fetoplacental ratios and offspring survival. Pregnant RIAKI dams also had albuminuria and less renal megalin in the proximal tubule brush border than shams, with renal subcapsular fibrosis and higher diastolic blood pressure. Growth-restricted offspring had a reduced GFR as older adults, with evidence of metabolic inefficiency in male offspring; this correlated with reduced renal AngII levels in female offspring from recovered RIAKI pairings. However, the blood pressures of 6-month-old offspring were unaffected by parental RIAKI.
Conclusions: Our mouse model demonstrated a causal relationship among RIAKI, gestational risk, and developmental programming of the adult-onset offspring GFR and metabolic dysregulation despite parental recovery.
Introduction
Acute kidney injury (AKI) is defined by sudden-onset renal cell injury and functional impairment. AKI is diagnosed in over 13 million people globally each year, but subclinical and, consequently, additional undiagnosed cases are common, especially in otherwise healthy adults ( Petejova and Martinek, 2014 ; Zou et al., 2022 ). Although the recovery of renal function occurs within days to weeks, AKI has been linked to delayed-onset consequences, including increased risk of chronic kidney disease (CKD), mortality, stroke, dementia, hypertension, and fetal growth restriction, costing the United States healthcare system a total of $90 billion per year ( Miyazawa et al., 2002 ; Kelly, 2003 ; Deng et al., 2004 ; Kim et al., 2006 ; Liu et al., 2008 ; James et al., 2011 ; Chawla et al., 2014 ; Makris and Spanou, 2016 ; Silver and Chertow, 2017 ; Hebert et al., 2023 ). CKD, which may result from AKI, further impairs reproductive function, fertility, and germ cell quality in both sexes ( Holley and Schmidt, 2013 ; Palant et al., 2017 ; Dumanski and Ahmed, 2019 ; Lundy and Vij, 2019 ).
Most investigations into the consequences of AKI have focused on older adults; however, multiple etiologies of AKI primarily affect young and otherwise healthy individuals, including sepsis, certain drugs, COVID-19, and rhabdomyolysis ( Abdel-Kader and Palevsky, 2009 ; Makris and Spanou, 2016 ; Gameiro et al., 2020 ; Nadim et al., 2020 ; Turgut et al., 2023 ). Because of their younger age and expected years of life, reproductive-age AKI survivors are a large population at risk for late AKI-initiated disease. Rhabdomyolysis-induced acute kidney injury (RIAKI) occurs following muscular injury induced by blunt objects (as in assault, motor vehicle crashes, and earthquakes), blast injury, drug-induced obtundation, or physical overexertion ( Elterman et al., 2015 ; Hummel et al., 2016 ; Stewart et al., 2016 ). The damaged muscle releases myoglobin into the bloodstream, which is reabsorbed by the renal proximal tubule by megalin and cubilin after glomerular filtration ( Gburek et al., 2003 ). Upon entry into proximal tubule cells, free iron from myoglobin forms reactive oxygen species and causes cell death. Currently, there is no treatment for RIAKI except fluid resuscitation ( Lameire et al., 2008 ; Kodadek et al., 2022 ).
As men have an overall higher incidence of AKI, outcomes in women are under-characterized ( Schiffl, 2020 ; Loutradis et al., 2021 ). However, women with renal disease are at risk for adverse maternal and fetal outcomes ( Harville et al., 2019 ; Suarez et al., 2019 ; Garovic et al., 2022 ); specifically, a history of recovered AKI in women who later get pregnant increases the risk for preeclampsia and small-for-gestational-age babies ( Tangren et al., 2017 ; Piccoli et al., 2018 ; Tangren et al., 2018 ). The maternal kidney controls the systemic vascular tone and blood pressure through the renin–angiotensin system (RAS), primarily via the balance of vasoconstrictive angiotensin II (AngII) to vasodilating factors, which can also influence the localized placental RAS and fetal RAS driving nephrogenesis ( Woods et al., 2001 ; Woods et al., 2004 ; Lumbers et al., 2019 ). In addition to the limited studies on maternal outcomes following recovered AKI, no studies have addressed offspring beyond the neonatal stage or the mechanisms of heritable and long-term disease ( Perico et al., 2018 ). Using a well-characterized mouse model of RIAKI, a form of AKI often observed in people of childbearing age ( Wilson et al., 1967 ; Wei et al., 2011 ; Matsushita et al., 2021 ), we hypothesized that recovered parental RIAKI would result in poor pregnancy outcomes, impaired fetal growth, and later-onset offspring disease.
All procedures described below were approved by the Institutional Animal Care and Use Committee of the Portland Veterans Administration Health Care System (protocol #4514-20). The mice were given ad libitum access to 5L0D PicoLab Laboratory Rodent Diet (LabDiet), a balanced nutritional rodent chow, at all points of the experiment, including during urinary collection in metabolic cages.
Rhabdomyolysis model
RIAKI was performed as previously described by our group and others ( Wilson et al., 1967 ; Wei et al., 2011 ; Matsushita et al., 2021 ). Male and female 8–12-week-old C57BL/6 mice were subjected to water deprivation for 4 h prior to the procedure. Rhabdomyolysis was induced under isoflurane anesthesia by an anterior thigh intramuscular injection of 50% glycerol in saline (8 mL/kg, half-dose per side), while sham animals were untreated.
Glomerular filtration rate
The glomerular filtration rate (GFR; uL/min/100 g body weight) was assessed at 24 h and 2 weeks after rhabdomyolysis as the transcutaneous quantification of fluorescein isothiocyanate (FITC)–sinistrin elimination ( Scarfe et al., 2018 ). A region on the abdominal section of the mouse was depilated, and a fluorescence detector (MediBeacon) was applied. After a 5-min baseline reading, FITC–sinistrin (50 μL, 35 mg/mL) was injected into the retro-orbital venous plexus. Elimination was quantified while the mouse was active and awake for 90 min using MediBeacon software. The GFR was calculated using the half-life of elimination.
Mating and fertility assessment
Both parents may contribute to fetal and placental development, and paternal metabolic health has been shown to influence pregnancy ( Galaviz-Hernandez et al., 2019 ; Eberle et al., 2020 ); therefore, the sires and dams were subjected to RIAKI and recovery before breeding. After documenting a return to a normal GFR 2 weeks after rhabdomyolysis, timed sham male/sham female and RIAKI male/RIAKI female pairings were established. Female mice were exposed to male mouse urine for 72 h before breeding to stimulate and synchronize estrus before breeding in single-matched pairs; the formation of a vaginal plug was classified as gestational day (GD) 0.5 ( Whitten, 1956 ; Hebert et al., 2021 ). The time from pair introduction to birth was considered as the days to conception.
Perinatal mortality and offspring weights
Dams were visually monitored twice daily. The initial litter size was the number of pups present at birth. Perinatal death was calculated as the percentage of initial litter that died or went missing (and presumed ingested) between birth and weaning. Pups were weaned at 3 weeks old and were housed with sex-matched siblings throughout longitudinal assessments. Weights were measured at one-week intervals from birth to 12 weeks (young adulthood cohort). The older adulthood cohort was also weighed at 6 months.
Pregnancy and placental efficiency assessments
At GD 16.5 (term GD 19.5), urine was collected from pregnant dams for 24 h in metabolic cages. After the GFR was measured, the dams were euthanized by injecting a lethal dose of tribromoethanol. The maternal kidney, heart, placenta, and fetus were all weighed fresh; maternal blood (plasma), kidneys, heart, and placentas, and fetal liver and kidney were collected. All samples were flash-frozen in tubes dipped in liquid nitrogen except the maternal heart and left maternal kidney, which were fixed overnight in 4% paraformaldehyde for histology. Fetoplacental sufficiency was calculated as the ratio of fetal weight/placental weight ( Wilson and Ford, 2001 ).
Young and older adult offspring assessments
The GFR was measured in 12-week-old (young adult) and 6-month-old (older adult) offspring of RIAKI and sham pairings, as described above, followed by euthanasia by a lethal injection of tribromoethanol. Plasma and laterally bisected right kidneys were flash-frozen after saline perfusion, with hearts and left kidneys perfusion-fixed via the left ventricular apex with 4% paraformaldehyde and collected for histology. Kidneys, hearts, and body weights were all measured.
Blood pressure measurements
Systolic and diastolic blood pressure, mean arterial pressure (MAP), and pulse were measured before measuring the GFR in GD-16.5 dams and 6-month-old offspring using a computerized tail-cuff system (Hatteras Systems, MC4000 Blood Pressure Analysis System). The 6-month-old mice were acclimated to the measuring system for 3 days, with recordings obtained at the same time each day; no statistical difference was observed in day-to-day measurements. Each animal underwent a cycle of 10 preliminary and 10 experimental measurements daily.
Urine was collected for 24 h in urine collection cups pretreated with a protease inhibitor, starting immediately after an animal’s recovery from experimental anesthesia, and measured. Urine was centrifuged at 1,500 g for 15 min to remove particulates; the supernatant was stored at −80°. The total urine protein was measured using a bicinchoninic acid (BCA) kit (Pierce BCA, 23227, Thermo Fisher). Equal volumes of urine were loaded for urine gel electrophoresis; gels were stained with Coomassie Blue and imaged. Urine albumin (Albuwell M, 1011, Ethos Biosciences) and retinol-binding protein 4 (RBP4; ab202404, Abcam) levels were measured by enzyme-linked immunosorbent assay (ELISA).
Plasma analysis
After collection, blood was centrifuged at 2,000 g for 15 min to obtain plasma, which was separated and frozen at −80°. The blood urea nitrogen (BUN) level was measured in diluted plasma samples using a colorimetric detection kit (EIABUN, Thermo Fisher).
Quantification of renal brush border proteins
Flash-frozen renal tissue was digested to isolate the renal proximal tubule brush border membranes, as previously described ( Biber et al., 1981 ). In brief, the homogenized mouse renal cortex was homogenized in an isosmotic medium with ethylene glycol tetraacetic acid (EGTA) and precipitated twice with magnesium chloride and centrifuged to obtain a high yield of brush border membranes. The total protein was quantified using a BCA kit. For megalin detection by Western blot, 3 ug of isolated protein was run on 3%–8% Tris–acetate gels (NuPAGE, Invitrogen) at 170 V for 60 min, transferred to polyvinylidene difluoride (PVDF) membranes, and blocked as previously described ( Matsushita et al., 2021 ). The membranes were exposed to a megalin primary antibody (1:1,000; Thermo Fisher) overnight at 4°, washed, and horseradish peroxidase-conjugated secondary antibody-treated (1:5,000; Thermo Fisher) for 2 h at room temperature before final washes and development with SuperSignal West Dura (Thermo Fisher). Images were captured using the ChemiDoc Imaging System (Bio-Rad). The brush border AngII level was determined by AngII ELISA (S-1133, BMA Biomedicals). ACE2 activity was measured following incubation with intramolecularly quenched synthetic ACE2-specific substrate Mca-APK(Dnp) (AnaSpec), as previously described ( Wysocki et al., 2014 ).
Statistical analysis
Experimental numbers were determined by a priori power analyses (power of 80%) using the sample size calculator designed by Wang and Ji (2020) and supported by R (v4.0, package pwr, pwr.anova.test, and pwr.t.test). Statistical analysis was performed using Prism 10.0 (GraphPad). Two-group comparisons were performed using Student’s t-test, with Welch’s correction as appropriate. Multiple-group comparisons were performed with ANOVA (or two-way ANOVA in the case of before–after comparisons in the same mice), with Tukey’s HSD test as appropriate. Risk ratios were calculated with α = 0.05. Statistical significance was considered to be p < 0.05. Figures present all data as dots with means and standard error. Any unmarked comparisons in the figures are considered statistically not significant.
Rhabdomyolysis causes recoverable acute kidney injury in male and female mice
First, we characterized RIAKI and its resolution in male and female mice. Here, 24 h after the induction of rhabdomyolysis, the male and female mice had a reduced GFR compared to the sex-matched controls; the GFR returned to baseline by 2 weeks post-rhabdomyolysis ( Figure 1A ). The blood urea nitrogen level was increased 24 h following rhabdomyolysis but returned to baseline within 2 weeks ( Figure 1B ), with similar results observed in urine albumin concentrations ( Figure 1C ). RBP4 is a small protein associated with proximal tubule dysfunction when found in the urine ( Norden et al., 2014 ); it significantly increased 24 h following rhabdomyolysis ( Figure 1D ). These data confirm the development of AKI after glycerol injection and recovery from AKI before breeding.
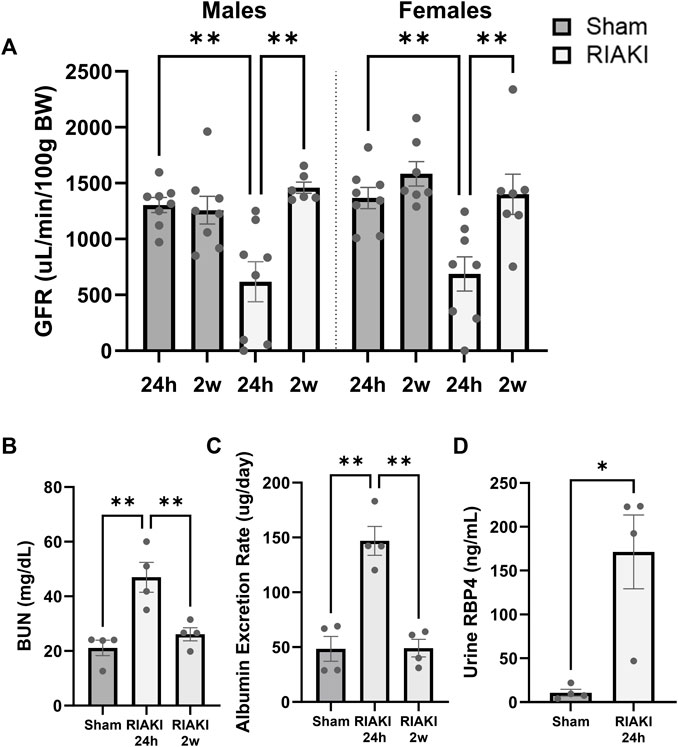
Figure 1 . The glycerol injection model of rhabdomyolysis-induced acute kidney injury (RIAKI) caused transient renal functional impairment, which was reversed by 2 weeks. (A) Glomerular filtration rate (GFR; uL/min/100 g body weight [BW]) of the control versus RIAKI male (left) and female (right) mice. The GFR is significantly reduced after rhabdomyolysis but recovers 2 weeks post-injury. (B) High levels of blood urea nitrogen (BUN) and (C) urinary albumin were observed following RIAKI. BUN and urinary albumin levels returned to baseline by 2 weeks. (D) The urinary retinol-binding protein 4 (RBP4) level, a marker of proximal tubule dysfunction, was increased significantly 24 h following RIAKI. One-way ANOVA with post hoc tests for multiple-group analyses and the t-test for two-group analyses were conducted. *: p < 0.05. **: p < 0.01.
RIAKI affects fetal and perinatal outcomes but not fertility
Next, we evaluated the effect of RIAKI on fertility and perinatal outcomes. Sham and RIAKI matings demonstrated similar time to conception after pairing ( Figure 2A ). No difference in weights between dams on GD 16.5 (term 19.5) was observed (sham: 34.03 g ± 2.69 g; RIAKI: 34.62 ± 3.00 g; NS). Additionally, initial litter sizes at birth were the same in both groups ( Figure 2B ). However, pups from RIAKI pairings had a 3.14 ± 0.50 times higher relative risk of death in the perinatal (birth to weaning) period than pups from sham pregnancies, with approximately ¼ of pups from RIAKI pairing litters dying before weaning ( Figure 2C ). Although fetal weights ( Figure 2D ) and placental weights ( Figure 2E ) were not significantly different between the groups, the ratio of the fetal weight to placental weight was 16.8% lower in pups from RIAKI pairs, indicating placental insufficiency and fetal growth restriction ( Figure 2F ). Birthweights were 5.0% lower in RIAKI pups, and pups grew less quickly in the first week, although weights caught up by 2 weeks of age and remained similar through 12 weeks ( Figures 2G , 4 ). This difference in fetal weight was not mediated by sex; males and females from RIAKI pairings were significantly smaller at 1 week ( Figure 2H ). We conclude that RIAKI pairings did not experience reduced fertility but resulted in growth-restricted pups with higher perinatal mortality than those from sham pairings.
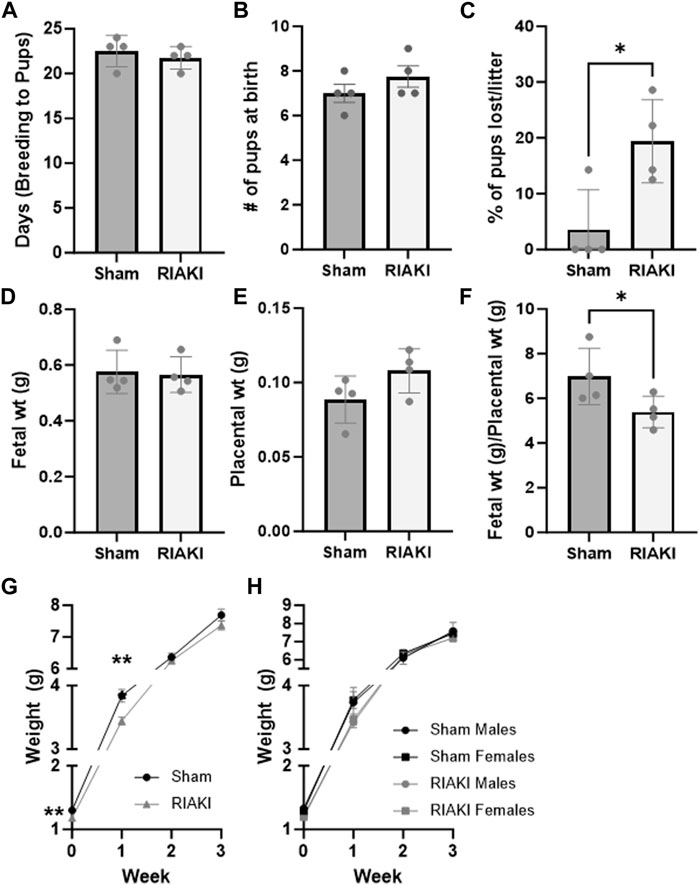
Figure 2 . Recovered parental RIAKI causes fetal growth restriction but does not affect fertility. RIAKI did not affect fertility, as measured by (A) the number of days from pair introduction to birth and (B) litter sites. (C) More pups from RIAKI pairings died in the perinatal period than pups from sham pairings. Neither (D) fetal nor (E) placental weight was significantly different, but (F) placental sufficiency (fetal weight/placental weight) decreased in pups from RIAKI pairings. (G) Pups from RIAKI pairs were born smaller and remained smaller in the first week than pups from shams. By 2 weeks, the weights of the pups were not different between groups. (H) Fetal sex was not a factor in differences. Litters: n = 4. (D–F) Presented as the average per litter. (A–G) T-test for two-group analyses. (H) One-way ANOVA with post hoc tests. *: p < 0.05. **: p < 0.01.
Recovered parental RIAKI causes abnormal pregnancy
To evaluate the influence of recovered RIAKI on maternal and intrauterine physiology, we evaluated renal function, blood pressure, and select components of the intra-renal RAS at GD 16.5. This time point is considered late in pregnancy when the fetus is still in a period of rapid growth but after the placenta has reached its maximum development ( Coan et al., 2004 ). Although renal functional recovery was evident in parents 2 weeks following RIAKI, the GFR in RIAKI-bred dams was reduced compared to sham dams ( Figure 3A ). Masson’s trichrome-stained histological sections showed evidence of subcapsular fibrosis in RIAKI dams that was absent in sham dams ( Figure 3B ). Furthermore, 24-h urine protein excretion was increased in RIAKI dams 24 h following rhabdomyolysis, which was resolved in 2 weeks, but proteinuria returned in these dams by GD 16.5, particularly low-molecular weight proteinuria ( Figure 3C ). Measuring the urine albumin content by ELISA confirmed albuminuria in pregnancy following recovered AKI seven times greater than that of sham dams ( Figure 3D ); this was driven by the albumin concentration and not urine volume ( Figure 3E ). A Western blot for megalin on proteins isolated from the renal brush border revealed lower megalin expression in RIAKI dams than in shams ( Figure 3F ; Supplementary Figure S1 ), supporting a conclusion of unrecovered or pregnancy-exacerbated proximal tubule damage following RIAKI. Assessment of renin–angiotensin system factors in RIAKI dams revealed a reduced angiotensin-converting enzyme 2 (ACE2) urine:tissue activity ratio ( Figure 3G ), suggesting RAS imbalance in the kidney. Dams showed no change in systolic pressure, but diastolic blood pressure was elevated in recovered RIAKI dams ( Figures 3H, I ). Together, these data indicate that pregnancies in recovered RIAKI dams are complicated by renal dysfunction caused by fibrosis and loss of tubular function, accompanied by alterations in blood pressure.
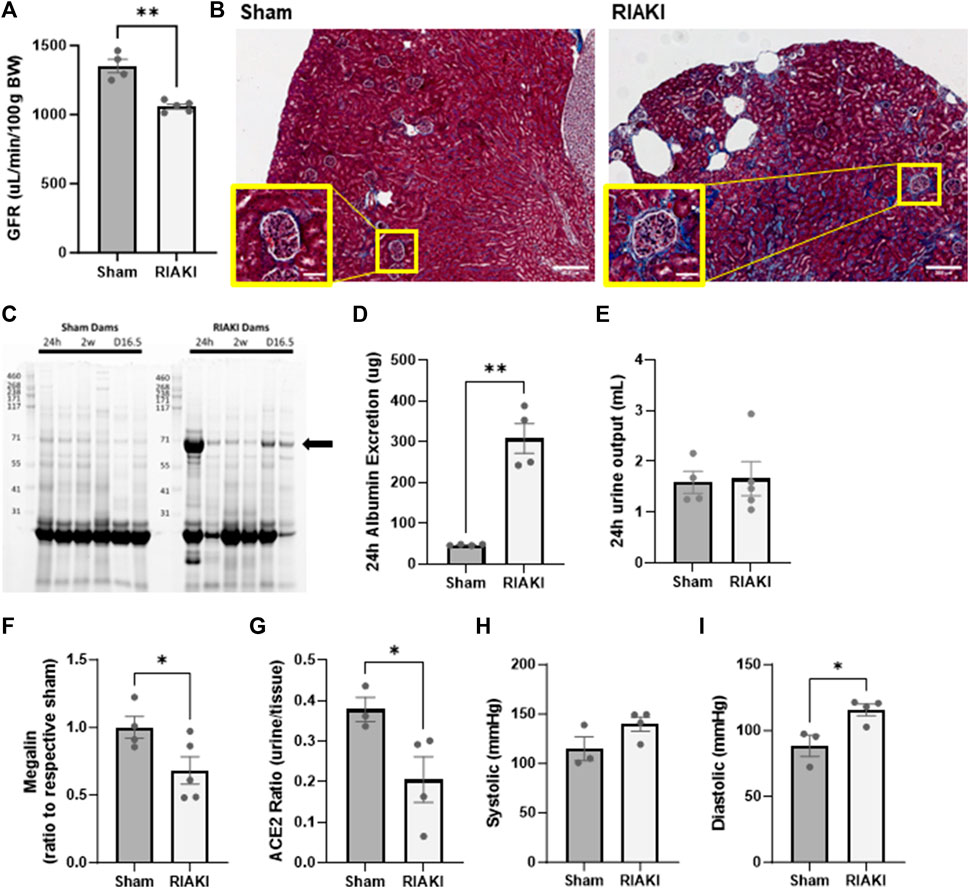
Figure 3 . Exposure to recovered RIAKI results in intrapregnancy renal functional and histological derangement at gestational day (GD) 16.5. (A) The GFR was selectively induced in recovered RIAKI-exposed dams, accompanied by (B) new subcapsular fibrosis (see insets). (C) RIAKI dams also exhibited low-molecular weight proteinuria in urine gels. The marked band was suspected to be albumin; we confirmed that the total urinary excretion of albumin over 24 h was elevated (D) . Urine excretion amounts were not different between groups over 24 h (E) . Assessment of (F) tubular megalin confirms that recovered RIAKI was correlated with the reduction in tubular megalin levels. (G) The ratio of urine to tissue ACE2 activity is reduced, confirming a change in renal renin–angiotensin system (RAS) components. No change was observed in (H) systolic blood pressure during late-term pregnancy, but (I) diastolic blood pressure was higher in recovered RIAKI dams. T-test for two groups. *: p < 0.05. **: p < 0.01.
Offspring of recovered RIAKI parents develop renal dysfunction and gain weight in adulthood
Next, to determine the inter-generational impact of parental AKI, we performed a longitudinal evaluation of the surviving offspring. Young adult offspring (12 weeks) of RIAKI dams did not have a significantly lower GFR than offspring of shams ( Figure 4A ; p = 0.12), a trend that did not change when data were separated by sex ( Figure 4B ). However, by middle age (6 months), the GFR was 17.4% lower in RIAKI adult offspring by group ( Figure 4C ) in both sexes ( Figure 4D ). Males from RIAKI parents gained more weight between young adulthood and middle age than shams, while females were not significantly different based on parentage ( Figure 4E ); this is reflected by a steeper weight gain slope, with males differing significantly ( p = 0.005, Figure 4F ). These data suggest that offspring may be developmentally programmed for adult-onset renal dysfunction by parental recovered RIAKI despite not being exposed to RIAKI themselves; moreover, male offspring of RIAKI pairings may be metabolically challenged in later life.
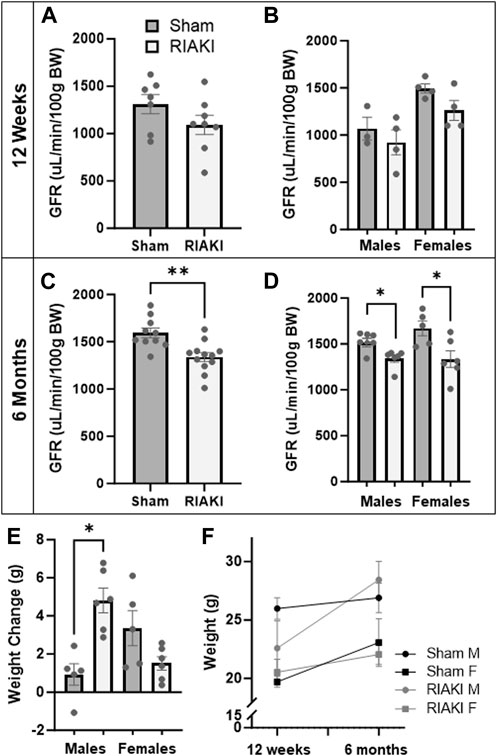
Figure 4 . Adult offspring of recovered RIAKI parents develop renal dysfunction with age. (A) The GFR of 12-week-old offspring was not significantly different, nor was (B) sex a factor. (C) By 6 months old, the GFR was reduced in all RIAKI offspring, (D) regardless of sex. (E) Between young (12 weeks) and middle (6 months) adulthood, males from RIAKI pairings gained significantly more weight than sham males, (F) with significantly steeper weight gain slopes (male slopes p = 0.005). T-test for two groups and one-way ANOVA with post hoc tests for multiple comparisons. *: p < 0.05. **: p < 0.01.
Perturbations of the RAS in early adulthood are not reflected in older adult blood pressures
Lastly, we evaluated the components of the intra-renal RAS in offspring. Measurements of renal AngII levels in sham and RIAKI offspring at 12 weeks old were not significantly different based on parentage alone ( Figure 5A ), but females from RIAKI dams had 43.6% lower AngII levels than female offspring of sham dams ( Figure 5B ). No apparent impact on blood pressure metrics was observed at 6 months: systolic ( Figure 5C ) and diastolic blood pressures ( Figure 5D ) were unchanged when analyzed with or without regard to separation by sex. This suggests that while the vasoconstrictive AngII level is reduced in female pups from RIAKI pairings in early adulthood, no changes in adult blood pressure are evident in RIAKI offspring.
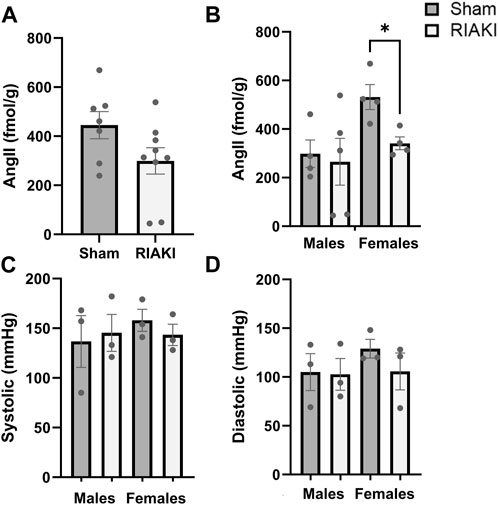
Figure 5 . RAS but not blood pressure is affected in adult offspring of sham and RIAKI parents. (A) In 12-week-old offspring, AngII kidney tissue content varied by breeding pairing and (B) offspring sex. Females from sham pairings had higher AngII levels than males; female offspring of RIAKI had lower AngII levels than sham females. However, (C) systolic and (D) diastolic blood pressures of 6-month-old offspring were not significantly different. T-test for two groups and one-way ANOVA with post hoc tests. *: p < 0.05.
In an AKI model relevant to reproductive health, we show that parents who have recovered from AKI nonetheless cause immediate and lifelong risks to their offspring. Despite recovery from AKI, we found that these dams develop new kidney disease during pregnancy, accompanied by increased blood pressure and an altered intra-renal RAS. Adult offspring of these dams developed delayed-onset renal and metabolic abnormalities. These findings likely resulted from parental systemic or renal disease, not reproductive system failure, since parental RIAKI did not alter fertility: RIAKI- and sham-exposed parents produced the same number of pups at the same interval. However, the offspring of RIAKI parents were small for their gestational age, more likely to die, and gained weight less rapidly than those from sham parents. This was accompanied by placental insufficiency, as measured by the fetal-to-placental weight ratio near term. Clinical studies have demonstrated an association between prior AKI and preeclampsia ( Tangren et al., 2017 ; Tangren et al., 2018 ). Furthermore, using the renal ischemia–reperfusion injury AKI model, Gillis et al. (2021) first characterized recovered AKI-induced fetal growth restriction with normal fertility and described impaired placental blood flow and altered antepartum maternal hemodynamics and renal sodium handling. Our findings corroborate those of Gillis et al.; we use a similar model of AKI that occurs in humans of reproductive age. Additionally, our study adds a novel understanding of the connection of the antepartum maternal kidney to the uterine environment to connect maternal and offspring disease pathogenesis.
In our AKI model, we investigated the maternal renal function and the intra-renal RAS, which plays a role in sodium and hemodynamic regulation ( Pohl et al., 2010 ; Bullen et al., 2023 ). The evaluation of antepartum maternal renal function, a principal determinant of the intrauterine environment, revealed surprising late-pregnancy GFR loss accompanied by tubular proteinuria and renal fibrosis. The observation of tubular proteinuria was striking and may be an important mechanistic insight. Pre-pregnancy RIAKI was accompanied by urine RBP4 elevation. RBP4 is a megalin ligand ( Norden et al., 2014 ) and, in the presence of tubular proteinuria, confirms the impairment of megalin-dependent function in the proximal tubule ( Bullen et al., 2023 ). The recurrence of GFR loss in pregnancy was coupled with reduced megalin expression, perhaps suggesting incomplete or maladaptive repair in the proximal tubule following acute kidney injury despite the outward appearance of recovery. As angiotensinogen and AngII bind and are internalized by megalin, and brush border ACE2 distribution is regulated by megalin ( Pohl et al., 2010 ), we evaluated renal ACE2 activity and AngII content. Our AngII findings were inconclusive ( Supplementary Figure S2 ), but antepartum megalin loss was accompanied by a reduced ratio of urine–brush border ACE2 activity, which we speculate may be a compensatory effect to attempt to conserve proximal tubule ACE2 levels. These data suggest that lasting or recurring disruption of megalin function by RIAKI dysregulates the intra-renal RAS in pregnancy.
Normal pregnancy relies on a balance of factors to preserve cardiovascular function, many of which are controlled by the kidney [reviewed by Touyz and Montezano (2018) ]. We observed diastolic blood pressure elevation in recovered RIAKI dams. We speculate that elevated blood pressure may result from RAS imbalance or increased renal (or plasma) AngII levels affecting the vascular tone. Further mechanistic study should focus on untangling the interactions between renal megalin and ACE2 during pregnancy and the resultant effects on systemic AngII blood pressure, sodium homeostasis, and fetal development.
Some of our findings may be seen as echoing aspects of human preeclampsia, in which albuminuria, hypertension, and RAS abnormalities are associated with fetal growth restriction and poor fetal outcomes. In distinction from preeclampsia, however, we did not find significantly elevated maternal systolic blood pressure. Tubular proteinuria, as opposed to glomerular proteinuria, and the absence of glomerular lesions on kidney sections argue against the development of endotheliopathy, which is a principal underpinning of preeclampsia. Therefore, the syndrome observed in our mouse model more closely resembles observations in mild or subclinical renal impairment: combined systolic and diastolic hypertension and endotheliopathy are not present during such pregnancies, but the risk of preterm birth and fetal growth restriction is increased ( Hladunewich et al., 2016 ). Since tight control of diastolic pressure during pregnancy in patients with hypertension is effective at controlling overall maternal hypertension ( Magee et al., 2015 ), and the treatment of maternal hypertension may improve fetal and maternal outcomes ( Dimitriadis et al., 2023 ), our preclinical study and related work may ultimately have translational implications for prenatal screening and care.
Since adverse pregnancies can program offspring for adult-onset disease ( Barker et al., 1989 ; Barker, 1990 ; Newnham, 2007 ; Rodrguez-Rodrguez et al., 2018 ; Lu and Hu, 2019 ), we considered long-term developmental programming consequences of parental RIAKI. We followed the offspring of RIAKI and sham pairings through adulthood. At 6 months old, we observed no change in blood pressure in the offspring of RIAKI-exposed parents, but they demonstrated a reduced GFR regardless of sex and altered weight gain exclusively in males. Although young adult offspring showed normal renal function, the renal angiotensin II level was reduced in the progeny of RIAKI parents, which may be an early warning of future renal dysfunction. This was entirely driven by the female sex: the female offspring of RIAKI parents halved their AngII level. Other studies of RAS balance have found that estrogen stimulates ACE2 expression and that males with CKD have lower ACE2 glomerular expression than females ( Shoemaker et al., 2019 ; Maksimowski et al., 2020 ). The observed reduction in AngII levels in young adult females might represent a compensatory response to preventing higher blood pressure, plasma sodium levels, or GFR. Alterations in the RAS in female offspring may help avoid some of the fate that befalls their male siblings.
Our study has limitations. First, our mouse model findings may not fully extend to human diseases. Because both paternal and maternal influence may determine fetal development, we studied parings in which both parents were exposed to sham or RIAKI. Subsequently, we cannot exclude paternal involvement in the observed effects or the necessity of exposing both parents to RIAKI. Further studies are necessary to determine whether maternal RIAKI is sufficient. We are currently investigating an isolated maternal post-RIAKI model with no paternal or fetal RIAKI influence to expand our studies. Similarly, we cannot exclude rearing behavior or efficient nutrient availability during the peripartum period as a cause of adult disease; further study will be required to elucidate the role of this vital component in development. The weight change observed in the adult male offspring of RIAKI parents is a valuable observation; although we do not currently know whether this is due to edema or obesity, both would point toward dysfunctional programming during development. Metabolic studies of offspring are planned to differentiate the causes of weight gain. Finally, blood pressures were measured in awake mice using tail plethysmography, which may have lacked sensitivity to detect subtle changes in blood pressure resulting from prior parental AKI. Although our studies were powered to examine the differences based on the GFR, the results generated by our blood pressure studies are hypothesis-generating and promising pilot data for future studies.
We conclude that parental recovered RIAKI causes impaired fetal development and maternal antepartum kidney disease reflected in megalin loss and an impaired intra-renal RAS. These data demonstrate both similarities and differences with characteristics of preeclampsia, a major clinical problem. Our data also implicate parental RIAKI in adult kidney disease of the offspring, suggesting a novel potential mechanism underpinning the developmental origins of the disease.
Data availability statement
The raw data supporting the conclusion of this article will be made available by the authors, without undue reservation.
Ethics statement
The animal study was approved by the Institutional Animal Care and Use Committee of the Portland Veterans Administration Health Care System. The study was conducted in accordance with the local legislation and institutional requirements.
Author contributions
JH: conceptualization, data curation, formal analysis, funding acquisition, investigation, methodology, project administration, visualization, writing–original draft, and writing–review and editing. YF: investigation, writing–original draft, and writing–review and editing. JE: data curation, investigation, writing–original draft, and writing–review and editing. MN: investigation, writing–original draft, and writing–review and editing. TG: investigation, project administration, writing–original draft, and writing–review and editing. NA: formal analysis, investigation, visualization, writing–original draft, and writing–review and editing. SG: conceptualization, methodology, supervision, writing–original draft, and writing–review and editing. MH: conceptualization, formal analysis, funding acquisition, investigation, methodology, project administration, resources, supervision, writing–original draft, and writing–review and editing.
The authors declare that financial support was received for the research, authorship, and/or publication of this article. This work was supported by the United States Department of Veterans Affairs, Veterans Health Administration, Office of Research and Development, Biomedical Laboratory Research and Development (VA Merit Award #101BX004288 to MH), and the United States Department of Defense (W81XWH2010196 to MH). JH was supported by the National Center for Advancing Translational Sciences, National Institutes of Health, through Grant Award Number TL1TR002371.
Acknowledgments
The authors thank Dr. Helen Liu of the OHSU APOM Research Core for her assistance in sectioning renal tissue and performing Masson’s trichrome stains.
Conflict of interest
The authors declare that the research was conducted in the absence of any commercial or financial relationships that could be construed as a potential conflict of interest.
Publisher’s note
All claims expressed in this article are solely those of the authors and do not necessarily represent those of their affiliated organizations, or those of the publisher, the editors, and the reviewers. Any product that may be evaluated in this article, or claim that may be made by its manufacturer, is not guaranteed or endorsed by the publisher.
Author disclaimer
The content is solely the responsibility of the authors and does not represent the official views of the US Department of Veterans Affairs, the US Government, or the NIH.
Supplementary material
The Supplementary Material for this article can be found online at: https://www.frontiersin.org/articles/10.3389/fphys.2024.1357932/full#supplementary-material
Abdel-Kader K., Palevsky P. M. (2009). Acute kidney injury in the elderly. Clin. Geriatr. Med. 25 (3), 331–358. doi:10.1016/j.cger.2009.04.001
PubMed Abstract | CrossRef Full Text | Google Scholar
Barker D. J. (1990). The fetal and infant origins of adult disease. BMJ Clin. Res. 301 (6761), 1111. doi:10.1136/bmj.301.6761.1111
Barker D. J., Osmond C., Golding J., Kuh D., Wadsworth M. E. (1989). Growth in utero , blood pressure in childhood and adult life, and mortality from cardiovascular disease. BMJ Clin. Res. 298 (6673), 564–567. doi:10.1136/bmj.298.6673.564
Biber J., Stieger B., Haase W., Murer H. (1981). A high yield preparation for rat kidney brush border membranes Different behaviour of lysosomal markers. Biochimica Biophysica Acta (BBA) - Biomembr. 647 (2), 169–176. doi:10.1016/0005-2736(81)90243-1
Bullen A. L., Fregoso A., Ascher S. B., Shlipak M. G., Ix J. H., Rifkin D. E. (2023). Markers of kidney tubule dysfunction and major adverse kidney events. Nephron. 147, 713–716. doi:10.1159/000531946
Chawla L. S., Amdur R. L., Shaw A. D., Faselis C., Palant C. E., Kimmel P. L. (2014). Association between AKI and long-term renal and cardiovascular outcomes in United States veterans. Clin. J. Am. Soc. Nephrol. 9 (3), 448–456. doi:10.2215/CJN.02440213
Coan P. M., Ferguson-Smith A. C., Burton G. J. (2004). Developmental dynamics of the definitive mouse placenta assessed by stereology. Biol. reproduction 70 (6), 1806–1813. doi:10.1095/biolreprod.103.024166
Deng J., Hu X., Yuen P. S., Star R. A. (2004). Alpha-melanocyte-stimulating hormone inhibits lung injury after renal ischemia/reperfusion. Am. J. Respir. Crit. Care Med. 169 (6), 749–756. doi:10.1164/rccm.200303-372OC
Dimitriadis E., Rolnik D. L., Zhou W., Estrada-Gutierrez G., Koga K., Francisco R. P. V., et al. (2023). Pre-eclampsia. Nat. Rev. Dis. Prim. 9 (1), 8. doi:10.1038/s41572-023-00417-6
CrossRef Full Text | Google Scholar
Dumanski S. M., Ahmed S. B. (2019). Fertility and reproductive care in chronic kidney disease. J. Nephrol. 32 (1), 39–50. doi:10.1007/s40620-018-00569-9
Eberle C., Kirchner M. F., Herden R., Stichling S. (2020). Paternal metabolic and cardiovascular programming of their offspring: a systematic scoping review. PLoS One 15 (12), e0244826. doi:10.1371/journal.pone.0244826
Elterman J., Zonies D., Stewart I., Fang R., Schreiber M. (2015). Rhabdomyolysis and acute kidney injury in the injured war fighter. J. Trauma Acute Care Surg. 79 (4), S171–S174. doi:10.1097/TA.0000000000000572
Galaviz-Hernandez C., Sosa-Macias M., Teran E., Garcia-Ortiz J. E., Lazalde-Ramos B. P. (2019). Paternal determinants in preeclampsia. Front. Physiology 9 (1870), 1870. doi:10.3389/fphys.2018.01870
Gameiro J., Fonseca J. A., Outerelo C., Lopes J. A. (2020). Acute kidney injury: from diagnosis to prevention and treatment strategies. J. Clin. Med. 9 (6), 1704. doi:10.3390/jcm9061704
Garovic V. D., Dechend R., Easterling T., Karumanchi S. A., McMurtry Baird S., Magee L. A., et al. (2022). Hypertension in pregnancy: diagnosis, blood pressure goals, and pharmacotherapy: a scientific statement from the American heart association. Hypertension 79 (2), e21–e41. doi:10.1161/HYP.0000000000000208
Gburek J., Birn H., Verroust P. J., Goj B., Jacobsen C., Moestrup S. K., et al. (2003). Renal uptake of myoglobin is mediated by the endocytic receptors megalin and cubilin. Am. J. Physiology-Renal Physiology 285 (3), F451–F458. doi:10.1152/ajprenal.00062.2003
Gillis E. E., Brands M. W., Sullivan J. C. (2021). Adverse maternal and fetal outcomes in a novel experimental model of pregnancy after recovery from renal ischemia-reperfusion injury. J. Am. Soc. Nephrol. 32 (2), 375–384. doi:10.1681/ASN.2020020127
Harville E. W., Catov J., Lewis C. E., Bibbins-Domingo K., Gunderson E. P. (2019). Pre-pregnancy kidney function and subsequent adverse pregnancy outcomes. Pregnancy Hypertens. 15, 195–200. doi:10.1016/j.preghy.2019.01.011
Hebert J. F., Funahashi Y., Hutchens M. P. (2023). Harm! foul! How acute kidney injury SHReDDs patient futures. Curr. Opin. Nephrol. Hypertens. 32 (2), 165–171. doi:10.1097/MNH.0000000000000864
Hebert J. F., Millar J. A., Raghavan R., Romney A., Podrabsky J. E., Rennie M. Y., et al. (2021). Male fetal sex affects uteroplacental angiogenesis in growth restriction mouse model. Biol. Reproduction 104 (4), 924–934. doi:10.1093/biolre/ioab006
Hladunewich M. A., Melamed N., Bramham K. (2016). Pregnancy across the spectrum of chronic kidney disease. Kidney Int. 89 (5), 995–1007. doi:10.1016/j.kint.2015.12.050
Holley J. L., Schmidt R. J. (2013). Changes in fertility and hormone replacement therapy in kidney disease. Adv. Chronic Kidney Dis. 20 (3), 240–245. doi:10.1053/j.ackd.2013.01.003
Hummel K., Gregory A., Desai N., Diamond A. (2016). Rhabdomyolysis in adolescent athletes: review of cases. Phys. Sportsmed. 44 (2), 195–199. doi:10.1080/00913847.2016.1170582
James M. T., Ghali W. A., Knudtson M. L., Ravani P., Tonelli M., Faris P., et al. (2011). Associations between acute kidney injury and cardiovascular and renal outcomes after coronary angiography. Circulation 123 (4), 409–416. doi:10.1161/CIRCULATIONAHA.110.970160
Kelly K. J. (2003). Distant effects of experimental renal ischemia/reperfusion injury. J. Am. Soc. Nephrol. 14 (6), 1549–1558. doi:10.1097/01.asn.0000064946.94590.46
Kim D. J., Park S. H., Sheen M. R., Jeon U. S., Kim S. W., Koh E. S., et al. (2006). Comparison of experimental lung injury from acute renal failure with injury due to sepsis. Respiration 73 (6), 815–824. doi:10.1159/000095588
Kodadek L., Carmichael S. P., Seshadri A., Pathak A., Hoth J., Appelbaum R., et al. (2022). Rhabdomyolysis: an American association for the surgery of trauma critical care committee clinical consensus document. Trauma Surg. Acute Care Open 7 (1), e000836. doi:10.1136/tsaco-2021-000836
Lameire N., Van Biesen W., Hoste E., Vanholder R. (2008). The prevention of acute kidney injury: an in-depth narrative review Part 1: volume resuscitation and avoidance of drug- and nephrotoxin-induced AKI. NDT Plus 1, 392–402. doi:10.1093/ndtplus/sfn162
Liu M., Liang Y., Chigurupati S., Lathia J. D., Pletnikov M., Sun Z., et al. (2008). Acute kidney injury leads to inflammation and functional changes in the brain. J. Am. Soc. Nephrol. 19 (7), 1360–1370. doi:10.1681/ASN.2007080901
Loutradis C., Pickup L., Law J. P., Dasgupta I., Townend J. N., Cockwell P., et al. (2021). Acute kidney injury is more common in men than women after accounting for socioeconomic status, ethnicity, alcohol intake and smoking history. Biol. Sex Differ. 12 (1), 30. doi:10.1186/s13293-021-00373-4
Lu H. Q., Hu R. (2019). Lasting effects of intrauterine exposure to preeclampsia on offspring and the underlying mechanism. AJP Rep. 9 (3), e275–e291. doi:10.1055/s-0039-1695004
Lumbers E. R., Delforce S. J., Arthurs A. L., Pringle K. G. (2019). Causes and consequences of the dysregulated maternal renin-angiotensin system in preeclampsia. Front. Endocrinol. 10, 563. doi:10.3389/fendo.2019.00563
Lundy S. D., Vij S. C. (2019). Male infertility in renal failure and transplantation. Transl. Androl. Urol. 8 (2), 173–181. doi:10.21037/tau.2018.07.16
Magee L. A., von Dadelszen P., Rey E., Ross S., Asztalos E., Murphy K. E., et al. (2015). Less-tight versus tight control of hypertension in pregnancy. N. Engl. J. Med. 372 (5), 407–417. doi:10.1056/NEJMoa1404595
Makris K., Spanou L. (2016). Acute kidney injury: definition, Pathophysiology and clinical phenotypes. Clin. Biochem. Rev. 37 (2), 85–98.
PubMed Abstract | Google Scholar
Maksimowski N., Williams V. R., Scholey J. W. (2020). Kidney ACE2 expression: implications for chronic kidney disease. PLoS One 15 (10), e0241534. doi:10.1371/journal.pone.0241534
Matsushita K., Mori K., Saritas T., Eiwaz M., Funahashi Y., Nickerson M., et al. (2021). Cilastatin ameliorates rhabdomyolysis-induced AKI in mice. J. Am. Soc. Nephrol. 32 (10), 2579–2594. doi:10.1681/ASN.2020030263
Miyazawa S., Watanabe H., Miyaji C., Hotta O., Abo T. (2002). Leukocyte accumulation and changes in extra-renal organs during renal ischemia reperfusion in mice. J. Lab. Clin. Med. 139 (5), 269–278. doi:10.1067/mlc.2002.122832
Nadim M. K., Forni L. G., Mehta R. L., Connor M. J., Liu K. D., Ostermann M., et al. (2020). COVID-19-associated acute kidney injury: consensus report of the 25th Acute Disease Quality Initiative (ADQI) Workgroup. Nat. Rev. Nephrol. 16 (12), 747–764. doi:10.1038/s41581-020-00356-5
Newnham J. P. (2007). The developmental origins of health and disease (DOHaD) – why it is so important to those who work in fetal medicine. Ultrasound Obstet. Gynecol. 29, 121–123. doi:10.1002/uog.3938
Norden A. G., Lapsley M., Unwin R. J. (2014). Urine retinol-binding protein 4: a functional biomarker of the proximal renal tubule. Adv. Clin. Chem. 63, 85–122. doi:10.1016/b978-0-12-800094-6.00003-0
Palant C. E., Amdur R. L., Chawla L. S. (2017). Long-term consequences of acute kidney injury in the perioperative setting. Curr. Opin. Anesthesiol. 30 (1), 100–104. doi:10.1097/ACO.0000000000000428
Perico N., Askenazi D., Cortinovis M., Remuzzi G. (2018). Maternal and environmental risk factors for neonatal AKI and its long-term consequences. Nat. Rev. Nephrol. 14 (11), 688–703. doi:10.1038/s41581-018-0054-y
Petejova N., Martinek A. (2014). Acute kidney injury due to rhabdomyolysis and renal replacement therapy: a critical review. Crit. Care 18 (3), 224. doi:10.1186/cc13897
Piccoli G. B., Alrukhaimi M., Liu Z.-H., Zakharova E., Levin A.World Kidney Day Steering Committee (2018). Women and kidney diseases: questions unanswered and answers unquestioned. Kidney Int. Rep. 3 (2), 225–235. doi:10.1016/j.ekir.2018.01.001
Pohl M., Kaminski H., Castrop H., Bader M., Himmerkus N., Bleich M., et al. (2010). Intrarenal renin angiotensin system revisited: role of megalin-dependent endocytosis along the proximal nephron. J. Biol. Chem. 285 (53), 41935–41946. doi:10.1074/jbc.M110.150284
Rodrguez-Rodrguez P., Ramiro-Cortijo D., Reyes-Hernndez C. G., Lopez de Pablo A. L., Gonzalez M. C., Arribas S. M. (2018). Implication of oxidative stress in fetal programming of cardiovascular disease. Front. Physiol. 9, 602. doi:10.3389/fphys.2018.00602
Scarfe L., Schock-Kusch D., Ressel L., Friedemann J., Shulhevich Y., Murray P., et al. (2018). Transdermal measurement of glomerular filtration rate in mice. J. Vis. Exp. 140, 58520. doi:10.3791/58520
Schiffl H. (2020). Gender differences in the susceptibility of hospital-acquired acute kidney injury: more questions than answers. Int. Urology Nephrol. 52 (10), 1911–1914. doi:10.1007/s11255-020-02526-7
Shoemaker R., Tannock L. R., Su W., Gong M., Gurley S. B., Thatcher S. E., et al. (2019). Adipocyte deficiency of ACE2 increases systolic blood pressures of obese female C57BL/6 mice. Biol. Sex. Differ. 10 (1), 45. doi:10.1186/s13293-019-0260-8
Silver S. A., Chertow G. M. (2017). The economic consequences of acute kidney injury. Nephron 137 (4), 297–301. doi:10.1159/000475607
Stewart I. J., Faulk T. I., Sosnov J. A., Clemens M. S., Elterman J., Ross J. D., et al. (2016). Rhabdomyolysis among critically ill combat casualties: associations with acute kidney injury and mortality. J. Trauma Acute Care Surg. 80 (3), 492–498. doi:10.1097/TA.0000000000000933
Suarez M. L. G., Kattah A., Grande J. P., Garovic V. (2019). Renal disorders in pregnancy: Core curriculum 2019. Am. J. Kidney Dis. 73 (1), 119–130. doi:10.1053/j.ajkd.2018.06.006
Tangren J. S., Powe C. E., Ankers E., Ecker J., Bramham K., Hladunewich M. A., et al. (2017). Pregnancy outcomes after clinical recovery from AKI. J. Am. Soc. Nephrol. 28 (5), 1566–1574. doi:10.1681/ASN.2016070806
Tangren J. S., Wan M., Adnan W. A. H., Powe C. E., Ecker J., Bramham K., et al. (2018). Risk of preeclampsia and pregnancy complications in women with a history of acute kidney injury. Hypertension 72 (2), 451–459. doi:10.1161/HYPERTENSIONAHA.118.11161
Touyz R. M., Montezano A. C. (2018). Angiotensin-(1-7) and vascular function: the clinical context. Hypertension 71 (1), 68–69. doi:10.1161/HYPERTENSIONAHA.117.10406
Turgut F., Awad A. S., Abdel-Rahman E. M. (2023). Acute kidney injury: medical causes and pathogenesis. J. Clin. Med. 12 (1), 375. doi:10.3390/jcm12010375
Wang X., Ji X. (2020). Sample size estimation in clinical research: from randomized controlled trials to observational studies. Chest 158 (1), S12–S20. doi:10.1016/j.chest.2020.03.010
Wei Q., Hill W. D., Su Y., Huang S., Dong Z. (2011). Heme oxygenase-1 induction contributes to renoprotection by G-CSF during rhabdomyolysis-associated acute kidney injury. Am. J. Physiology - Ren. Physiology 301 (1), F162–F170. doi:10.1152/ajprenal.00438.2010
Whitten W. K. (1956). Modification of the oestrous cycle of the mouse by external stimuli associated with the male. J. Endocrinol. 13 (4), 399–404. doi:10.1677/joe.0.0130399
Wilson D. R., Thiel G., Arce M. L., Oken D. E. (1967). Glycerol induced hemoglobinuric acute renal failure in the rat. 3. Micropuncture study of the effects of mannitol and isotonic saline on individual nephron function. Nephron 4 (6), 337–355. doi:10.1159/000179594
Wilson M. E., Ford S. P. (2001). Comparative aspects of placental efficiency. Reprod. Suppl. 58, 223–232. doi:10.1530/biosciprocs.16.0016
Woods L. L., Ingelfinger J. R., Nyengaard J. R., Rasch R. (2001). Maternal protein restriction suppresses the newborn renin-angiotensin system and programs adult hypertension in rats. Pediatr. Res. 49 (4), 460–467. doi:10.1203/00006450-200104000-00005
Woods L. L., Weeks D. A., Rasch R. (2004). Programming of adult blood pressure by maternal protein restriction: role of nephrogenesis. Kidney Int. 65 (4), 1339–1348. doi:10.1111/j.1523-1755.2004.00511.x
Wysocki J., Ortiz-Melo D. I., Mattocks N. K., Xu K., Prescott J., Evora K., et al. (2014). ACE2 deficiency increases NADPH-mediated oxidative stress in the kidney. Physiol. Rep. 2 (3), e00264. doi:10.1002/phy2.264
Zou C., Wang C., Lu L. (2022). Advances in the study of subclinical AKI biomarkers. Front. Physiol. 13, 960059. doi:10.3389/fphys.2022.960059
Keywords: acute kidney injury, pregnancy, developmental programming, renal function, rhabdomyolysis
Citation: Hebert JF, Funahashi Y, Emathinger JM, Nickerson MN, Groat T, Andeen NK, Gurley SB and Hutchens MP (2024) Parental recovered acute kidney injury causes prenatal renal dysfunction and fetal growth restriction with sexually dimorphic implications for adult offspring. Front. Physiol. 15:1357932. doi: 10.3389/fphys.2024.1357932
Received: 19 December 2023; Accepted: 20 March 2024; Published: 12 April 2024.
Reviewed by:
Copyright © 2024 Hebert, Funahashi, Emathinger, Nickerson, Groat, Andeen, Gurley and Hutchens. This is an open-access article distributed under the terms of the Creative Commons Attribution License (CC BY). The use, distribution or reproduction in other forums is permitted, provided the original author(s) and the copyright owner(s) are credited and that the original publication in this journal is cited, in accordance with accepted academic practice. No use, distribution or reproduction is permitted which does not comply with these terms.
*Correspondence: Jessica F. Hebert, [email protected]
† Present address: Megan N. Nickerson, Department of Anesthesiology, Perioperative and Pain Medicine, Stanford University School of Medicine
Disclaimer: All claims expressed in this article are solely those of the authors and do not necessarily represent those of their affiliated organizations, or those of the publisher, the editors and the reviewers. Any product that may be evaluated in this article or claim that may be made by its manufacturer is not guaranteed or endorsed by the publisher.
- Open access
- Published: 08 April 2024
Attributable mortality of acute kidney injury among critically ill patients with sepsis: a multicenter, retrospective cohort study
- Dong-Hui Wang 1 na1 ,
- Jin-Chao Zhao 2 na1 ,
- Xiu-Ming Xi 3 ,
- Yue Zheng 1 &
- Wen-Xiong Li 1
BMC Nephrology volume 25 , Article number: 125 ( 2024 ) Cite this article
70 Accesses
1 Altmetric
Metrics details
Sepsis and acute kidney injury (AKI) are common severe diseases in the intensive care unit (ICU). This study aimed to estimate the attributable mortality of AKI among critically ill patients with sepsis and to assess whether AKI was an independent risk factor for 30-day mortality.
The information we used was derived from a multicenter prospective cohort study conducted in 18 Chinese ICUs, focusing on septic patients post ICU admission. The patients were categorized into two groups: those who developed AKI (AKI group) within seven days following a sepsis diagnosis and those who did not develop AKI (non-AKI group). Using propensity score matching (PSM), patients were matched 1:1 as AKI and non-AKI groups. We then calculated the mortality rate attributable to AKI in septic patients. Furthermore, a survival analysis was conducted comparing the matched AKI and non-AKI septic patients. The primary outcome of interest was the 30-day mortality rate following the diagnosis of sepsis.
Out of the 2175 eligible septic patients, 61.7% developed AKI. After the application of PSM, a total of 784 septic patients who developed AKI were matched in a 1:1 ratio with 784 septic patients who did not develop AKI. The overall 30-day attributable mortality of AKI was 6.6% (95% CI 2.3 ∼ 10.9%, p = 0.002). A subgroup analysis revealed that the 30-day attributable mortality rates for stage 1, stage 2, and stage 3 AKI were 0.6% (95% CI −5.9 ∼ 7.2%, p = 0.846), 4.7% (95% CI −3.1 ∼ 12.4%, p = 0.221) and 16.8% (95% CI 8.1 ∼ 25.2%, p < 0.001), respectively. Particularly noteworthy was that stage 3 AKI emerged as an independent risk factor for 30-day mortality, possessing an adjusted hazard ratio of 1.80 (95% CI 1.31 ∼ 2.47, p < 0.001).
Conclusions
The overall 30-day attributable mortality of AKI among critically ill patients with sepsis was 6.6%. Stage 3 AKI had the most significant contribution to 30-day mortality, while stage 1 and stage 2 AKI did not increase excess mortality.
Peer Review reports
Sepsis is defined as a life-threatening organ dysfunction caused by the host’s dysregulated response to infection [ 1 ], leading to one-third to one-sixth of global deaths [ 2 , 3 , 4 ]. Acute kidney injury (AKI) is characterized by a sharp decline in renal function caused by multiple causes in a short time. The harmful inflammatory cascade reaction of sepsis may be related to AKI [ 5 , 6 ]. Sepsis is the leading cause of AKI in critically ill patients [ 7 ], and about 50% of septic patients are complicated with AKI [ 8 , 9 ]. Compared to other causes of AKI, sepsis-related AKI leads to higher mortality [ 5 ].
Determining the precise proportion of sepsis-related deaths caused by AKI is a challenging task, particularly considering the intricate condition of septic patients in the intensive care unit (ICU). As such, accurate determination of the percentage of sepsis-related deaths that are specifically attributable to AKI remains elusive. Estimating AKI’s impact on mortality in critically ill septic patients is crucial for planning clinical trials [ 10 ]. Vaara ST estimated that the 90-day absolute excess mortality caused by AKI in ICU patients was 8.6% [ 10 ]. According to Jiang Yijia’s report, new-onset AKI was found to contribute to excess 30-day mortality of 8.1% in general ICU patients [ 11 ]. It is worth noting that studies on AKI-attributable mortality vary significantly among different studies. So, what exactly is the AKI excess mortality in ICU septic patients? Therefore, we undertook a comprehensive secondary data analysis of a large prospective multicentre cohort study. Employing propensity score matching (PSM) analysis, we meticulously reduced confounding biases to provide a more accurate estimation of the excess mortality specifically attributed to AKI. Additionally, we assessed whether AKI emerged as an independent risk factor for 30-day mortality among septic patients in the ICU.
Materials and methods
Study patients.
The data for our study were derived from a prospective multicentre cohort study conducted by the China Critical Care Sepsis Trial (CCCST) working group. The study was carried out in 18 Chinese ICUs. The period was from 1 January 2014 to 31 August 2015. The CCCST study aimed to investigate the epidemiology and characteristics of critically ill patients with sepsis. Our study specifically targeted patients who met the sepsis 3.0 criteria [ 1 ] after ICU admission, thus being conclusively diagnosed with sepsis. The first day of diagnosis of sepsis was defined as the onset of sepsis. Following this diagnosis, patients were closely monitored for any signs of AKI that occurred within the first seven days. The exclusion criteria included: (1) patients already suffered from AKI of ICU admission; (2) AKI occurred before sepsis; (3) AKI occurred seven days later after the diagnosis of sepsis; (4) patients suffered chronic kidney disease (CKD), operated with nephrectomy or kidney transplantation; (5) patients received renal replacement therapy (RRT) for non-renal conditions; (6) missing data; (7) patients younger than 18 years of age. All participants or their close relatives had signed an informed consent form.
The included patients were categorized into two groups: those who developed AKI (AKI group) within seven days following a sepsis diagnosis and those who did not develop AKI (non-AKI group).
Definitions and endpoints
The definition of sepsis 3.0 was used to define sepsis [ 1 ]. For cases before 2016, this definition was applied retrospectively. Septic shock was defined as sepsis requiring vasopressors after adequate fluid resuscitation to maintain mean arterial pressure (MAP) ≥ 65 mmHg and blood lactate concentration ≥ 2 mmol/L [ 1 , 12 ]. The diagnosis and stage of AKI were based on the serum creatinine and urine output criteria proposed by Kidney Disease: Improving Global Outcomes (KDIGO) [ 13 ]. The definition of baseline creatinine was as follows: if at least five values were available, the median of all values from 6 months to 6 days before hospitalization was used; otherwise, the minimum value was five days before hospitalization [ 14 ]. If no creatinine was available before hospitalization or the emergency patient’s serum creatinine was abnormal at admission, baseline creatinine was estimated using the Modification of Diet in Renal Disease (MDRD) equation [ 15 ]. Attributable mortality of AKI was defined as the proportion of deaths that can be statistically attributed to AKI [ 10 , 11 , 16 , 17 ]. It was calculated by subtracting the mortality of matched septic patients without AKI from the mortality of matched septic patients with AKI (i.e., attributable mortality of AKI = Mortality in matched AKI patients–Mortality in matched non-AKI patients).
The primary endpoint was 30-day mortality after sepsis diagnosis. The secondary endpoint was the ICU length of stay (LOS), hospital LOS, ICU mortality, and hospital mortality.
Data collection
Data information included demographic characteristics [gender, age, and body mass index (BMI)], comorbidities [chronic obstructive pulmonary disease (COPD) or asthma, cardiovascular disease, hypertension, diabetes, cancer, and chronic liver disease], admission type (medical, surgical or emergency), and ICU diagnosis. The Acute Physiology and Chronic Health Evaluation II (APACHE II) score and the non-renal Sequential Organ Failure Assessment (SOFA) score were recorded on the sepsis diagnosis day. We collected clinical management data, including mechanical ventilation and renal replacement therapy (RRT), on the day of sepsis diagnosis. The mean arterial pressure (MAP) and the presence of septic shock were recorded daily within seven days of the definitive diagnosis of sepsis. We also recorded the baseline creatinine, the use of nephrotoxic drugs (angiotensin-converting enzyme inhibitors, aminoglycosides, nonsteroidal anti-inflammatory drugs, and vancomycin, et al.) [ 18 , 19 ], ICU LOS, hospital LOS, ICU mortality, 30-day mortality, and hospital mortality.
Statistical analysis
Statistics were analyzed using R 4.2.3. Continuous variables were presented as medians (interquartile range, IQR). Categorical variables are expressed as percentages. For comparing continuous data, we used the Mann-Whitney U test, and for categorical variables, the Chi-square test was utilized. A significance level of p < 0.05 was considered to indicate statistical significance. Applying PSM between septic patients with and without AKI to estimate the excess mortality attributed to AKI (Fig. 1 A). PSM was performed using the “matchit” package to balance non-renal variables on outcomes. This study used the nearest neighbor method with a caliper value of 0.06 of the standard deviation of the logit of the propensity score. The propensity score, which was based on baseline characteristics and clinical covariates, was used to adjust the differences between matched patients with and without AKI. The variables analyzed for PSM included age, gender, BMI, comorbidities, APACHE II score, non-renal SOFA score, baseline creatinine, mechanical ventilation, MAP, septic shock, and the use of nephrotoxic drugs. The variables used for propensity score matching models did not exhibit multicollinearity. The multicollinearity tests were presented in Supplemental Table S2 . We checked the covariate balance using standardized differences and considered values of over 25% indicative of meaningful differences. McNemar’s test was applied to sensitivity analysis [ 20 ]. Then, the 30-day mortality in matched septic patients with and without AKI was calculated separately. Subgroup analysis of matched septic patients with and without AKI was based on the AKI stage (Fig. 1 B). Attributable mortality was calculated separately for total and different stages of AKI. A 95% confidence interval (CI) for the attributable mortality difference was estimated by Newcombe’s method [ 21 ].
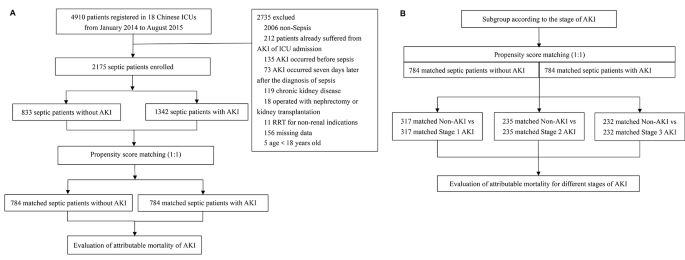
Study flow diagram ( A ) Flowchart of all the participants ( B ) Flowchart of the subgroup analysis. Abbreviations: ICU intensive care unit; AKI, acute kidney injury
Survival analysis was conducted on matched AKI and matched non-AKI septic patients. We used the Kaplan-Meier survival curve to compare the survival status of both groups and the log-rank test to compare their survival time and rate differences. We also used the multivariate Cox proportional hazard model to estimate the hazard ratio (HR) and 95% confidence interval (CI) for 30-day mortality after adjusting for age, gender, BMI, comorbidities, APACHE II score, non-renal SOFA score, baseline creatinine, mechanical ventilation, RRT, MAP, septic shock, and the use of nephrotoxic drugs.
Characteristics of the demographics and proportion of AKI
Of all 4910 patients, 2175 septic patients were included in this analysis (Table 1 ), which included 1342 (61.7%) cases of AKI that occurred within seven days after sepsis diagnosis (AKI group) and 833 (38.3%) cases who did not develop AKI (non-AKI group). Of the total, 1388 (63.8%) were male. The median (IQR) age was 64 (50–76) years. Patients in the AKI group had a higher BMI ( p < 0.05). The proportion of COPD or asthma, cardiovascular disease, diabetes, cancer, and chronic liver disease was similar between the two groups. Still, the proportion of hypertension in the AKI group was higher ( p < 0.05). 1213 (55.8%) were medical. The AKI group had higher APACHE II and non-renal SOFA scores ( p < 0.05) and was more likely to receive mechanical ventilation and suffer from septic shock ( p < 0.05). The septic patients in the AKI group had higher baseline creatinine and lower MAP ( p < 0.05). In the entire cohort, the median (IQR) ICU and hospital LOS were 8 (4–17) days and 18 (10–28) days, respectively. The 30-day mortality of AKI was 31.1%.
Among 1342 AKI patients, 474 (35.3%) were stage 1, 401 (29.9%) were stage 2, and 467 (34.8%) were stage 3. The 30-day mortality was 23.0%, 27.7%, and 42.2%, respectively ( p < 0.05). The baseline characteristics of different stages of AKI were shown in supplemental material Table S1 .
Attributable mortality of AKI in septic patients
Of the 2175 septic patients, 784 patients with AKI were matched 1:1 with 784 non-AKI patients, according to PSM. Characteristics and standardized differences of the matched patients in the two groups were shown in Table 2 . After PSM, no variable exhibited a significant imbalance. The histograms of propensity score before and after matching in cohorts with and without AKI were shown in Fig. 2 . The density plot of propensity score before and after matching in cohorts with and without AKI was presented in Supplemental material Fig. S1 . The Q-Q plots of the balance of the covariates were shown in Supplemental material Fig. S2 . The Jitter plot of the distribution of propensity scores was shown in Supplemental material Fig. S3 . The standardized difference before and after matching in cohorts with and without AKI was shown in Supplemental material Fig. S4 .
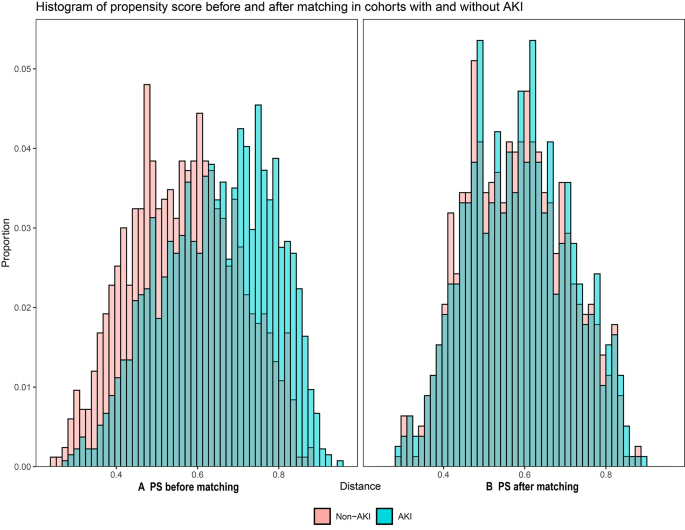
Histograms of propensity score before and after matching in cohorts with and without AKI ( A ) PS before matching ( B ) PS after matching. Abbreviations: PS, propensity score
The 30-day mortality of the matched septic patients with total AKI was 216 of 784 (27.6%) compared with 164 of 784 (21.0%) for septic patients without AKI ( p < 0.001). The 30-day attributable mortality of total AKI was 6.6% (95% CI 2.3 ∼ 10.9%, p = 0.002). Matched septic patients with AKI were subgrouped according to the AKI stage. A total of 317 patients with stage 1 AKI, 235 patients with stage 2 AKI, and 232 patients with stage 3 AKI were matched 1:1 with their controls without AKI, respectively. Subgroup analysis showed the 30-day attributable mortality of stage 1, stage 2, and stage 3 AKI was 0.6% (95% CI −5.9 ∼ 7.2%, p = 0.846), 4.7% (95% CI −3.1 ∼ 12.4%, p = 0.221) and 16.8% (95% CI 8.1 ∼ 25.2%, p < 0.001), respectively, as shown in Fig. 3 .
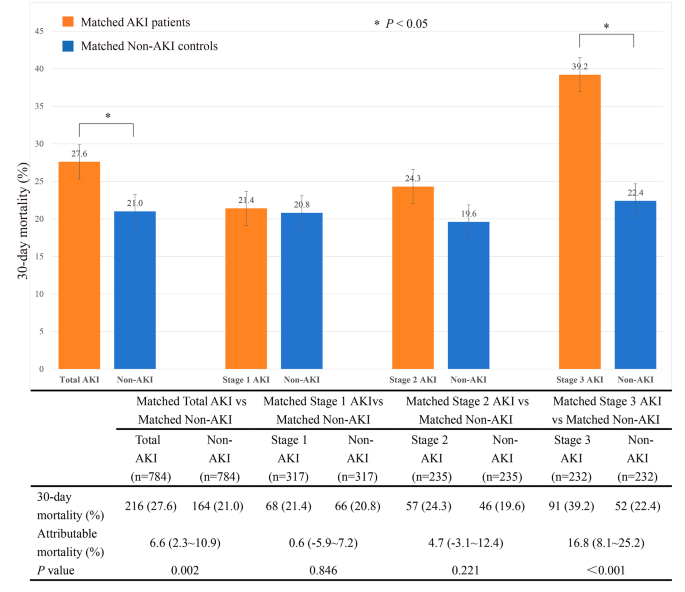
The attributable mortality of total AKI and different stages of AKI in subgroups Abbreviations: AKI, acute kidney injury
Sensitivity analysis
A sensitivity analysis was conducted to assess the impact of unmeasured covariates on the stability of results. The gamma parameter was used to measure the stability of results, which reflected the probability of differential assignment to treatment due to unobserved factors [ 22 , 23 ]. The effect estimate was considered robust to hidden bias if the statistical significance did not change until a large gamma. The results of this study showed that the estimation of 30-day attributable mortality of AKI was reliable for the hidden bias of unmeasured covariates, with a gamma coefficient of up to 3. The sensitivity analysis of 30-day attributable mortality results was shown in supplemental material Table S3 .
Survival analysis
In Fig. 4 A, the Kaplan-Meier curve revealed significantly higher 30-day mortality in the AKI group compared to the non-AKI group ( p < 0.001). Survival probabilities at the 30-day were 66.0% (95% CI 61.5 ∼ 70.8%) for non-AKI patients and 60.0% (95% CI 55.6 ∼ 64.8%) for AKI patients. Subgroup analysis demonstrated statistical significance between stage 1 and stage 3 AKI, as well as stage 2 and stage 3 AKI ( p < 0.001), as shown in Fig. 4 B. Survival probabilities at the 30-day were 68.4% (95% CI 61.9 ∼ 75.5%) for stage 1 AKI patients, 61.0% (95% CI 52.7 ∼ 70.7%) for stage 2 AKI patients, and 47.6% (95% CI 40.0 ∼ 56.5%) for stage 3 AKI patients.
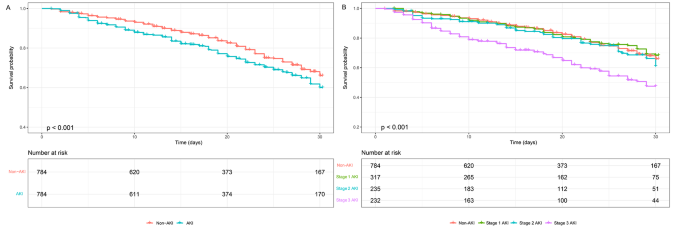
Kaplan-Meier survival analysis ( A ) Kaplan-Meier survival analysis comparing non-AKI and AKI groups ( B ) Kaplan-Meier survival analysis comparing non-AKI, stage 1 AKI, stage 2 AKI, and stage 3 AKI groups. p < 0.001 for all comparisons. The numbers of patients at risk at each time point were shown below the graph. Abbreviations: AKI, acute kidney injury
In our multivariate Cox regression adjusted model (Fig. 5 ), stage 3 AKI was associated with higher 30-day mortality, with an adjusted HR of 1.80 (95% CI 1.31 ∼ 2.47, p < 0.001). However, stage 1 and stage 2 AKI did not increase 30-day mortality, with adjusted HR of 0.90 (95% CI 0.68 ∼ 1.20, p = 0.482) and 1.24 (95% CI 0.91 ∼ 1.69, p = 0.168), respectively. Supplemental material Table S4 showed the multivariable Cox proportional hazard regression analysis for 30-day mortality stratified by AKI stage.
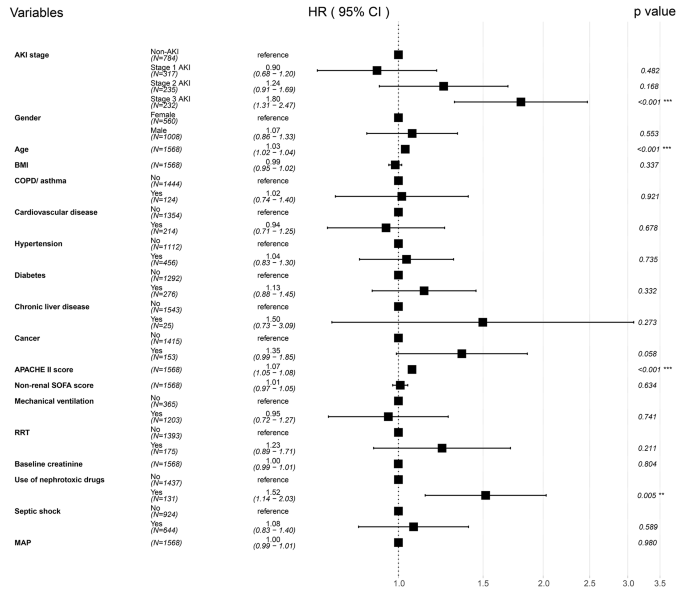
Forest plot of multivariable Cox proportional hazard regression analysis for 30-day mortality stratified by AKI stage. Abbreviations: AKI, acute kidney injury; BMI, body mass index; COPD, chronic obstructive pulmonary disease; APACHE II, acute physiologic and chronic health evaluation II; SOFA, sequential organ failure assessment; RRT, renal replacement therapy; MAP, mean arterial pressure
Sepsis is the leading cause of AKI [ 24 ]. Sepsis-related AKI is a common complication among critically ill patients and is associated with high morbidity and mortality [ 25 ]. One study that enrolled critically ill patients from 24 European countries reported an incidence rate of AKI in septic patients of 51%, and the mortality in ICU was 41% [ 8 ]. In contrast, another study that enrolled hospitalized patients across China reported a rate of 47.1% [ 9 ]. Recent evidence suggests that microvascular dysfunction, inflammation, and metabolic reprogramming may be the mechanisms leading to AKI in sepsis [ 7 ]. Since mortality rates are significantly influenced by patient demographics and the varying levels of care provided to critically ill patients with sepsis, the precise excess mortality attributed to AKI may differ considerably across different healthcare facilities. Calculating the attributable mortality of AKI would provide insight into the number of preventable deaths if AKI could be avoided [ 10 ]. We reported the proportion of deaths attributable to AKI in septic patients. This finding would help to calculate the sample size for future clinical trials of AKI in ICU septic patients. Our research found that the overall 30-day attributable mortality of AKI in septic patients was 6.6%. Subgroup analysis showed that the excess 30-day mortality of stage 3 AKI was 16.8%; stage 1 and stage 2 AKI did not increase the excess 30-day mortality, highlighting the importance of early recognition and treatment of AKI in septic patients. More aggressive treatment could help prevent the progression of AKI and improve the chances of survival, such as renal replacement therapy or immunomodulatory agents.
In 2014, Vaara ST conducted a study on 954 patients in a general ICU, which found that AKI lead to a 90-day absolute excess mortality rate of 8.6%. The study’s subgroup analysis revealed that patients in stage 1 AKI had a 90-day attributable mortality rate of 1.4%, while it was 12.0% for stage 2 AKI patients and a staggering 16.7% for stage 3 AKI patients [ 10 ]. A study performed by Jiang Yijia demonstrated that the attributable mortality for new-onset AKI in ICU patients was 8.1%. Interestingly, stage 1 AKI did not increase mortality, but stage 2 and stage 3 AKI had attributable mortality rates of 17.1% and 18.2%, respectively [ 11 ]. Our study discovered that stage 2 AKI did not increase the 30-day mortality rate of septic patients. This differed from the two similar studies, which included all general ICU patients. However, our study only focused on septic patients, which may account for the disparity in results. According to Wang Zhiyi’s research [ 26 ], the Medical Information Mart for Intensive Care (MIMIC) IV cohort, consisting of 15,610 patients, had an excess attributable mortality rate of 58.6% for AKI in critically ill patients with sepsis. In contrast, in the Wenzhou cohort, consisting of 1,341 patients, the excess attributable mortality was 44.6%. Wang Zhiyi’s results were higher than ours, probably because of the different approach used than the present study. They applied a directed acyclic graph (DAG) approach, which eliminated potential confounders and mediating variables, to calculate the attributable mortality rate for AKI. These studies all indicated that septic patients with stage 3 AKI had a poor prognosis. However, in a study meticulously conducted by Fujii in Japan, encompassing 424 septic patients and utilizing the sepsis 3.0 definition and KDIGO criteria, the anticipated impact of AKI on hospital mortality among the septic cohort remained elusive [ 27 ]. In contrast, our investigation revealed that stage 3 AKI notably elevated the 30-day mortality rate among septic patients. This divergence from the Japanese study could potentially be attributed to the relatively smaller sample size of septic patients, possibly obscuring any significant differences that may have otherwise been observed. The studies on the attributable mortality of AKI vary significantly among different studies, possibly due to the differences in race, healthcare resources availability, or clinical practice.
Our research demonstrated that the survival rate of stage 3 AKI was significantly lower, at only 47.6%. Cheyron’s study found that only severe AKI showed a significant association with excess attributable mortality in ICU patients diagnosed with liver cirrhosis [ 28 ]. Jiang YiJia reported in another study that new-onset AKI was a significant risk factor for 28-day mortality and persistent AKI was strongly linked to unfavorable outcomes [ 29 ]. Furthermore, our Cox regression analysis found that stage 3 AKI was an independent risk factor for 30-day mortality, with a mortality rate 0.80 times higher than that of non-AKI patients in the ICU. These findings suggested that early intervention and targeted treatment strategies may be critical in septic patients with stage 1–2 AKI, preventing kidney function deterioration from progressing to stage 3 AKI.
There were several highlights in this study. The data came from a large, multicenter, prospective clinical study in China, strengthening the results’ validity and generality. Secondly, we applied the definition of sepsis 3.0 and the KDIGO criteria for AKI. Thirdly, from a clinical perspective, our findings emphasize the critical need for healthcare professionals to remain vigilant when septic patients develop AKI. Preventing the progression to stage 3 AKI is imperative to ensure the best possible patient outcomes. However, there were also a few drawbacks in this study. It was imperative to acknowledge that the study under review was a retrospective secondary analysis, which may introduce unmeasured bias. The study engaged only those septic patients admitted to the ICU within seven days. However, additional research is required to comprehensively address cases where sepsis develops beyond this period. Consequently, additional observational studies are needed to gain a deeper and more comprehensive understanding of the findings.
The overall 30-day attributable mortality of AKI among critically ill patients with sepsis was 6.6%. Stage 3 AKI had the most significant contribution to 30-day mortality, while stage 1 and stage 2 AKI did not increase excess mortality. Stage 3 AKI was an independent risk factor for 30-day mortality.
Data availability
The datasets analyzed during the current study are available from the corresponding author upon reasonable request.
Abbreviations
- Acute kidney injury
Intensive care unit
- Propensity score matching
Body mass index
Chronic obstructive pulmonary disease
Chronic kidney disease
Acute physiologic and chronic health evaluation II
Sequential organ failure assessment
Renal replacement therapy
Mean arterial pressure
Length of stay
Interquartile range
Hazard ratio
Confidence interval
Singer M, Deutschman CS, Seymour CW, et al. The Third International Consensus definitions for Sepsis and septic shock (Sepsis-3). JAMA. 2016;315(8):801–10.
Article CAS PubMed PubMed Central Google Scholar
Evans L, Rhodes A, Alhazzani W, et al. Surviving Sepsis Campaign: International guidelines for Management of Sepsis and Septic Shock 2021. Crit Care Med. 2021;49(11):e1063–143.
Article PubMed Google Scholar
Fleischmann C, Scherag A, Adhikari NK, et al. Assessment of Global Incidence and Mortality of Hospital-treated Sepsis. Current estimates and limitations. Am J Respir Crit Care Med. 2016;193(3):259–72.
Article CAS PubMed Google Scholar
Fleischmann-Struzek C, Mellhammar L, Rose N, et al. Incidence and mortality of hospital- and ICU-treated sepsis: results from an updated and expanded systematic review and meta-analysis. Intensive Care Med. 2020;46(8):1552–62.
Bagshaw SM, Uchino S, Bellomo R, et al. Septic acute kidney injury in critically ill patients: clinical characteristics and outcomes. Clin J Am Soc Nephrol. 2007;2(3):431–9.
Yegenaga I, Hoste E, Van Biesen W, et al. Clinical characteristics of patients developing ARF due to sepsis/systemic inflammatory response syndrome: results of a prospective study. Am J Kidney Dis. 2004;43(5):817–24.
Peerapornratana S, Manrique-Caballero CL, Gómez H, et al. Acute kidney injury from sepsis: current concepts, epidemiology, pathophysiology, prevention and treatment. Kidney Int. 2019;96(5):1083–99.
Article PubMed PubMed Central Google Scholar
Vincent JL, Sakr Y, Sprung CL, et al. Sepsis in European intensive care units: results of the SOAP study. Crit Care Med. 2006;34(2):344–53.
Xu X, Nie S, Liu Z, et al. Epidemiology and clinical correlates of AKI in Chinese hospitalized adults. Clin J Am Soc Nephrol. 2015;10(9):1510–8.
Vaara ST, Pettilä V, Kaukonen KM, et al. The attributable mortality of acute kidney injury: a sequentially matched analysis. Crit Care Med. 2014;42(4):878–85.
Jiang YJ, Xi XM, Jia HM, et al. The attributable mortality of new-onset acute kidney injury among critically ill patients: a propensity-matched analysis based on a multicentre prospective cohort study. Int Urol Nephrol. 2022;54(8):1987–94.
Kamath S, Hammad Altaq H, Abdo T. Management of Sepsis and septic shock: what have we learned in the last two. Decades? Microorganisms. 2023;11(9):2231.
Palevsky PM, Liu KD, Brophy PD, et al. KDOQI US commentary on the 2012 KDIGO clinical practice guideline for acute kidney injury. Am J Kidney Dis. 2013;61(5):649–72.
Kashani K, Al-Khafaji A, Ardiles T, et al. Discovery and validation of cell cycle arrest biomarkers in human acute kidney injury. Crit Care. 2013;17(1):R25.
De Rosa S, Samoni S, Ronco C. Creatinine-based definitions: from baseline creatinine to serum creatinine adjustment in intensive care. Crit Care. 2016;20:69.
Wacholder S, Benichou J, Heineman EF, et al. Attributable risk: advantages of a broad definition of exposure. Am J Epidemiol. 1994;140(4):303–9.
Jia H-M, Jiang Y-J, Zheng X, et al. The attributable mortality of sepsis for acute kidney injury: a propensity-matched analysis based on multicenter prospective cohort study. Ren Fail. 2023;45(1):2162415.
Kwiatkowska E, Domański L, Dziedziejko V, et al. The mechanism of drug nephrotoxicity and the methods for preventing kidney damage. Int J Mol Sci. 2021;22(11):6109.
Popović B, Šutić I, Marković NB. NEPHROTOXIC DRUGS. Acta Med Croatica. 2016;70(4–5):309–14.
PubMed Google Scholar
Lee J, Little TD. A practical guide to propensity score analysis for applied clinical research. Behav Res Ther. 2017;98:76–90.
Newcombe RG. Improved confidence intervals for the difference between binomial proportions based on paired data. Stat Med. 1998;17(22):2635–50.
Rosenbaum PR. Sensitivity analysis for matched case-control studies. Biometrics. 1991;47(1):87–100.
Rosenbaum PR. Discussing hidden bias in observational studies. Ann Intern Med. 1991;115(11):901–5.
Hoste EA, Bagshaw SM, Bellomo R, et al. Epidemiology of acute kidney injury in critically ill patients: the multinational AKI-EPI study. Intensive Care Med. 2015;41(8):1411–23.
Bellomo R, Kellum JA, Ronco C, et al. Acute kidney injury in sepsis. Intensive Care Med. 2017;43(6):816–28.
Wang Z, Weng J, Yang J, et al. Acute kidney injury-attributable mortality in critically ill patients with sepsis. PeerJ. 2022;10:e13184.
Fujii T, Uchino S, Doi K, et al. Diagnosis, management, and prognosis of patients with acute kidney injury in Japanese intensive care units: the JAKID study. J Crit Care. 2018;47:185–91.
du Cheyron D, Bouchet B, Parienti JJ, et al. The attributable mortality of acute renal failure in critically ill patients with liver cirrhosis. Intensive Care Med. 2005;31(12):1693–9.
Jiang YJ, Xi XM, Jia HM, et al. Risk factors, clinical features and outcome of new-onset acute kidney injury among critically ill patients: a database analysis based on prospective cohort study. BMC Nephrol. 2021;22(1):289.
Download references
Acknowledgements
We greatly appreciated the support and assistance all the research centers in the CCCST working group offered. These 18 ICUs in China included Department of Critical Care Medicine, Fuxing Hospital, Capital Medical University, Beijing, China; Department of Critical Care Medicine, West China Hospital, Sichuan University, Sichuan, China; Medical Intensive Care Unit, Peking Union Medical College Hospital, Beijing, China; Department of Critical Care Medicine, Guangdong Geriatric Institute, Guangdong General Hospital, Guangdong, China; Department of Critical Care Medicine, The First Affiliated Hospital of China Medical University, Shenyang, China; Surgical Intensive Care Unit, Department of Anaesthesiology, ZhongShan Hospital, FuDan University, Shanghai, China; Intensive Care Unit, The First Hospital of Jilin University, Changchun, China; Department of Critical Care Medicine, China-Japan Friendship Hospital, Beijing, China; Department of Critical Care Medicine, Beijing Friendship Hospital, Capital Medical University, Beijing China; Surgical Intensive Care Unit, Beijing Chaoyang Hospital, Capital Medical University, Beijing, China; Department of Respiratory and Critical Care Medicine, Beijing Institute of Respiratory Medicine, Beijing Chaoyang Hospital, Capital Medical University, Beijing, China; Department of Critical Care Medicine, General Hospital of Ningxia Medical University, Ningxia, China; Department of Critical Care Medicine, Xiangya Hospital, Central South University, Changsha, China; Department of Critical Care Medicine, Beijing Tsinghua Changgung Hospital, Beijing, China; Department of Critical Care Medicine, Beijing Tongren Hospital, Capital Medical University, Beijing, China; Department of Critical Care Medicine, Peking University Third Hospital, Beijing, China; Surgical Intensive Care Unit, Xuanwu Hospital, Capital Medical University, Beijing, China and Department of Critical Care Medicine, Beijing Tiantan Hospital, Capital Medical University, Beijing, China.
This study was funded by the Beijing Municipal Science & Technology Commission (no. Z191100006619032).
Author information
Dong-Hui Wang and Jin-Chao Zhao contributed equally to this work.
Authors and Affiliations
Department of Surgical Intensive Care Unit, Beijing Chao-yang Hospital, Capital Medical University, 8 Gongren Tiyuchang Nanlu, Chaoyang District, 100020, Beijing, China
Dong-Hui Wang, Yue Zheng & Wen-Xiong Li
Department of Clinical Laboratory, Xiangyang No.1 People’ s Hospital, Hubei University of Medicine, 441000, Xiangyang, China
Jin-Chao Zhao
Department of Critical Care Medicine, Fuxing Hospital, Capital Medical University, Beijing, China
Xiu-Ming Xi
You can also search for this author in PubMed Google Scholar
Contributions
DH.W. collected and interpreted the data, drafted and revised the manuscript. JC.Z. and XM.X. performed material preparation, data collection, and analysis. Y. Z. provided critical comments to help revise the manuscript. WX. L. chaired the group and conceptualized and designed the study. All authors read and approved the final manuscript.
Corresponding authors
Correspondence to Yue Zheng or Wen-Xiong Li .
Ethics declarations
Ethics approval and consent to participate.
The study followed the ethical principles of the 1964 Declaration of Helsinki and was approved by the Hospital Human Ethics Committee of all participating ICUs. Fuxing Hospital, Capital Medical University Institutional Review Board granted the study. The registration number was ChiCTR-ECH-13003934, and the chief ethics number was 2013FXHEC-KY2018. All patients included in the study, or their close relatives were well informed about the study and a written informed consent was obtained.
Consent for publication
Not applicable.
Competing interests
The authors declare no competing interests.
Additional information
Publisher’s note.
Springer Nature remains neutral with regard to jurisdictional claims in published maps and institutional affiliations.
Electronic supplementary material
Below is the link to the electronic supplementary material.
Supplementary Material 1
Rights and permissions.
Open Access This article is licensed under a Creative Commons Attribution 4.0 International License, which permits use, sharing, adaptation, distribution and reproduction in any medium or format, as long as you give appropriate credit to the original author(s) and the source, provide a link to the Creative Commons licence, and indicate if changes were made. The images or other third party material in this article are included in the article’s Creative Commons licence, unless indicated otherwise in a credit line to the material. If material is not included in the article’s Creative Commons licence and your intended use is not permitted by statutory regulation or exceeds the permitted use, you will need to obtain permission directly from the copyright holder. To view a copy of this licence, visit http://creativecommons.org/licenses/by/4.0/ . The Creative Commons Public Domain Dedication waiver ( http://creativecommons.org/publicdomain/zero/1.0/ ) applies to the data made available in this article, unless otherwise stated in a credit line to the data.
Reprints and permissions
About this article
Cite this article.
Wang, DH., Zhao, JC., Xi, XM. et al. Attributable mortality of acute kidney injury among critically ill patients with sepsis: a multicenter, retrospective cohort study. BMC Nephrol 25 , 125 (2024). https://doi.org/10.1186/s12882-024-03551-9
Download citation
Received : 17 December 2023
Accepted : 19 March 2024
Published : 08 April 2024
DOI : https://doi.org/10.1186/s12882-024-03551-9
Share this article
Anyone you share the following link with will be able to read this content:
Sorry, a shareable link is not currently available for this article.
Provided by the Springer Nature SharedIt content-sharing initiative
- Attributable mortality
BMC Nephrology
ISSN: 1471-2369
- Submission enquiries: [email protected]
- General enquiries: [email protected]
- Open access
- Published: 30 April 2023
Optimization strategies of mesenchymal stem cell-based therapy for acute kidney injury
- Zhangning Fu 1 , 2 na1 ,
- Yifan Zhang 1 , 2 na1 ,
- Xiaodong Geng 2 , 3 ,
- Kun Chi 1 , 2 ,
- Chao Liu 4 ,
- Chengcheng Song 5 ,
- Guangyan Cai 2 ,
- Xiangmei Chen 2 &
- Quan Hong 2
Stem Cell Research & Therapy volume 14 , Article number: 116 ( 2023 ) Cite this article
1951 Accesses
3 Citations
Metrics details
Considering the high prevalence and the lack of targeted pharmacological management of acute kidney injury (AKI), the search for new therapeutic approaches for it is in urgent demand. Mesenchymal stem cells (MSCs) have been increasingly recognized as a promising candidate for the treatment of AKI. However, clinical translation of MSCs-based therapies is hindered due to the poor retention and survival rates as well as the impaired paracrine ability of MSCs post-delivery. To address these issues, a series of strategies including local administration, three-dimensional culture, and preconditioning have been applied. Owing to the emergence and development of these novel biotechnologies, the effectiveness of MSCs in experimental AKI models is greatly improved. Here, we summarize the different approaches suggested to optimize the efficacy of MSCs therapy, aiming at promoting the therapeutic effects of MSCs on AKI patients.
Introduction
Acute kidney injury (AKI), defined as a rapid increase in serum creatinine, decrease in urine output, or both, is a common clinical syndrome caused by multiple factors, including renal ischemia, sepsis, toxic effects from drugs, and pigment-related injury from myoglobin or hemoglobin [ 1 , 2 ]. According to a recent statistical report, AKI occurs in approximately 13.3 million people per year worldwide and the number is still increasing [ 1 , 3 ]. Except for several acute symptoms, AKI is strongly associated with subsequent chronic kidney disease (CKD) and end-stage kidney disease (ESKD) requiring necessary renal replacement therapy (RRT) or transplantation [ 4 , 5 , 6 ]. These sequelae place a significant financial burden not only on the patients and their families but also on the public healthcare services [ 7 ]. Current therapeutic approaches for AKI remain predominantly supportive and preventive, lacking in targeted pharmacological management [ 8 ]. In addition, since AKI often coexists with other syndromes such as heart failure, liver failure, and sepsis, AKI patients usually receive concomitant medications [ 1 , 9 ], which may consequently result in the increased risk of adverse effects. It is therefore imperative to develop more safe and effective strategies to treat and prevent the progression of AKI.
Mesenchymal stem cells (MSCs) are a kind of cells with robust self-renewal and multi-lineage differentiation potential, existing in many tissues including bone marrow, adipose tissue, umbilical cord blood, and placenta [ 10 ]. MSCs secret diverse cytokines, chemokines, growth factors, exosomes, and microvesicles (MVs), which exert cell proliferative, anti-fibrotic, anti-inflammatory, anti-apoptosis, angiogenic, regenerative, and immunomodulatory effects [ 11 , 12 , 13 , 14 ]. Besides the release of paracrine or endocrine factors, the therapeutic mechanism of MSCs therapy also involves direct cell-to-cell interactions [ 15 ]. Increasing evidence has shown the promising renal protective effects of MSCs in AKI [ 16 , 17 , 18 , 19 , 20 ]. However, there are still some limitations hindering the clinical success of this MSCs-based therapy, for example, the low engraftment, poor survival, and the impaired paracrine ability of MSCs after administration [ 21 ]. To overcome these obstacles, many innovative approaches have been explored in recent years. This review discusses these novel strategies in the setting of AKI.
Strategies for improving the therapeutic effects of MSCs
Advances in MSCs biology and bioengineering have shed light on new strategies that have the potential to address many of the limitations related to MSCs-based therapy. These strategies include the improvement in administration routes, the application of three-dimensional (3D) approaches, and the use of preconditioning methods.
Improvement in administration routes
For MSCs to exert their multiple therapeutic functions, a sufficient number of cells are required to be transferred to the sites of injury, which is the basis of MSCs treatment. Therefore, the choice of route of administration appears to be one of the most critical factors influencing the efficacy of MSCs-based therapy. However, no consensus has been reached on the best route for the administration of MSCs. For current preclinical and clinical trials, intravenous, intra-arterial and topical are the three commonly used routes (Fig. 1 ) [ 22 ].
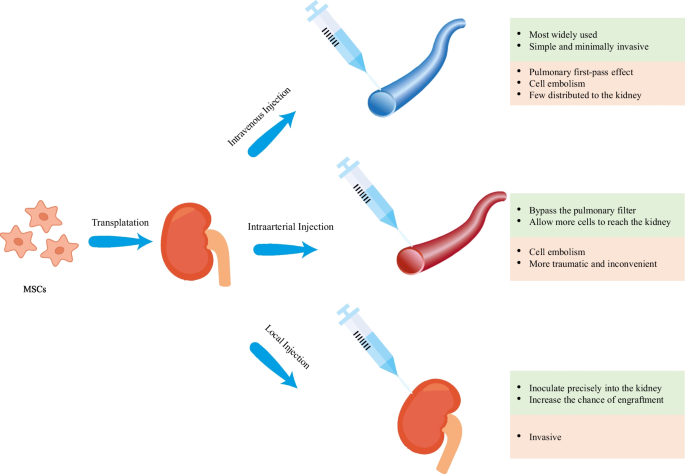
Common administration routes for MSCs transplantation in AKI models
Intravenous (IV) administration is the most widely used route in recent studies [ 22 ]. This route is a simple and minimally invasive way to deliver MSCs systematically into the animal models and human bodies. Despite these benefits, there is a great potential for MSCs to be trapped in the lungs, which is known as the pulmonary first-pass effect [ 23 , 24 ]. In addition to the lungs, liver is also indicated to be a concentrated gathering place of MSCs after IV injection [ 25 ], resulting in an inadequate therapeutic concentration in the kidney. Investigators have attempted to employ a higher dose of MSCs to solve this problem but this may increase the risk of adverse events such as pulmonary embolism and thrombotic complications like sinusoidal obstructive syndrome [ 26 , 27 , 28 , 29 ]. Intra-arterial (IA) administration has been proved to be more efficacious than IV administration and has been used as an alternative in some treatment indications [ 22 ]. Selective IA delivery of MSCs can bypass the pulmonary filter, improving the homing of MSCs and allowing more cells to reach the targeted tissue. However, this route also has the limitation of entailing the risk of cell embolism and is more traumatic as well as inconvenient in clinical practice [ 22 , 30 ]. Local administration of MSCs is more advantageous compared with IV and IA delivery and thus has become a new focus of current research [ 31 ]. MSCs can be inoculated precisely into the kidney via this route, increasing the chance of engraftment and enhancing their therapeutic potential. In addition, local injection avoids the lung barrier, decreasing the risk of lung infarction and mortality [ 24 , 30 ]. This injection method may be especially beneficial for kidney regeneration as it allows vast amounts of cells to be located at the site of interests. Several local delivery methods have been tested in the animal models of AKI and have shown encouraging results in recent years [ 31 , 32 , 33 , 34 , 35 , 36 , 37 , 38 , 39 , 40 , 41 , 42 , 43 ] (Table 1 ). Among them, renal cortex injection is more widely researched (Table 1 ), possibly because of its relatively strong operability and reversible injury to the kidney [ 44 ]. As shown in Table 1 , renal function recovery has been observed and the tubular injury has been ameliorated, with no identifiable safety concerns. However, since few studies have directly compared these different routes of MSC transplantation, additional research is needed to further explore the optimal routes of MSCs administration.
3D stem cell approaches for both culture and delivery
Aside from the delivery mode, several other factors such as the cell growth environment also impact the retention and viability of the stem cells [ 21 , 45 ]. Conventional approaches for MSCs culture are based on two-dimensional (2D) systems. Cells culture on these platforms often quickly undergoes senescence and a loss of cell functions [ 46 , 47 , 48 ]. To address these drawbacks, a beneficial 3D microenvironment is necessary to be designed to bridge the gap between the traditional culture system and the complex architecture in vivo. In addition, it is also worth noting that 3D approaches have become a frontier in stem cell delivery by virtue of their superior capability of promoting the survival and function of the transplanted cells [ 49 ]. Various methods have been developed to meet the demand for 3D cell culture and delivery over recent years. Broadly, these approaches can be categorized into scaffold-based (hydrogel-focused) and scaffold-free (spheroid culture) strategies (Fig. 2 ).
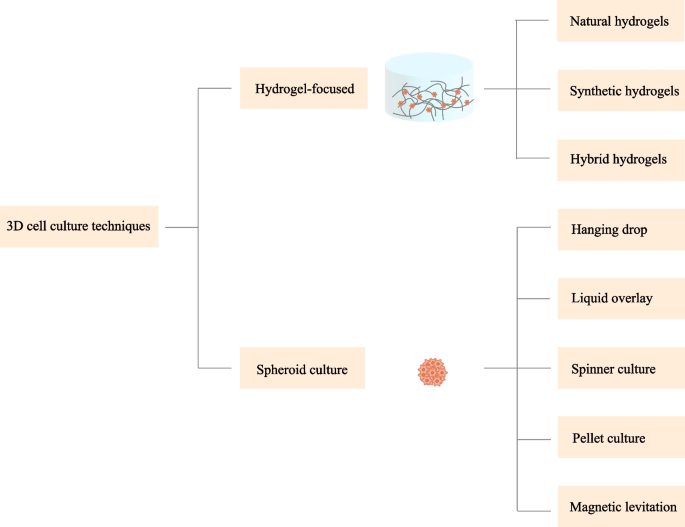
Technologies applied in 3D cell culture
Hydrogel-focused strategies
Hydrogels have been considered as one of the most promising candidates for 3D stem cell culture and delivery [ 50 , 51 ]. Hydrogels are chemically or physically cross-linked 3D porous polymeric networks characterized by high water content and tailorable mechanical, physical, and chemical properties [ 51 , 52 , 53 ]. The high moisture content and porous structure of hydrogels make it possible for the nutrients and metabolites to be transported in the networks [ 54 , 55 ]. Hydrogels in this way, can act as an artificial extracellular matrix (ECM) surrounding the cells, providing necessary conditions for cell–cell and cell–matrix interactions, which as a result influence the behaviors and functions of MSCs [ 21 ]. To date, a large number of hydrogels have been investigated to mimic the native microenvironment where the cells reside in vivo, ranging from natural to synthetic origin [ 21 ]. The utility of hydrogels as scaffolds in supporting the growth and function of the cells has also been demonstrated in many preclinical AKI models [ 32 , 39 , 42 , 56 ]. Below, we outline the categories of hydrogels and their corresponding properties.
Natural polymer hydrogels
Natural polymer-based hydrogels have attracted considerable attention over the past decade due to their good biocompatibility, biodegradability, and environmental friendliness [ 57 ]. Widely used natural polymers include agarose [ 58 ], alginate [ 59 ], chitosan [ 60 ], hyaluronic acid [ 61 ], collagen [ 62 ], gelatin [ 63 ], and fibrin [ 64 ], which can be further sub-classified into polysaccharide-based and protein-based hydrogels [ 48 ].
Agarose Agarose is a prominent marine polysaccharide extracted from agar or red seaweeds [ 58 ], possessing great biocompatibility, tunable mechanical characteristics, non-toxicity, non-immunogenicity, and thermo-reversible gelling properties [ 65 ]. This gel is made up of alternating residues of 1,3-linked β- d -galactopyranose and 1,4-linked 3,6-anhydro-α- l -galactopyranose [ 66 ]. Research works have shown that agarose can be used as culture scaffolds to enhance cell attachment and proliferation [ 55 ]. In addition, its thermally reversible characteristics offer great opportunities for its injection into the kidney with minimal invasiveness. However, agarose may be related to less enhanced cell functionality [ 65 ]. As agarose is seldom employed in current research of AKI, its applicability in renal tissues needs to be further studied.
Alginate The natural polymer alginate is a hydrophilic linear polysaccharide isolated from brown algae and certain bacteria, consisting of β-d-mannuronic acid (M) and α-l-guluronic acid (G) [ 59 ]. With the benign nature of cost-effectiveness, high biocompatibility, low cytotoxicity, and appropriate rheological properties, this soluble biopolymer is nowadays one of the most commonly employed bioinks in 3D bioprinting [ 67 ]. Prior to its use as a bioink, alginate has been extensively explored as a culture system and delivery vehicle for MSCs in the fields of regenerative medicine and tissue engineering [ 68 , 69 , 70 , 71 , 72 ]. Alginate also plays a significant role in the controlled release of the paracrine factors derived from MSCs [ 71 , 73 , 74 ]. However, alginate offers poor biodegradability and cell adhesive properties, which limit its potential applications [ 67 , 70 ]. Investigators are trying to overcome these limitations, and a recent work has indicated that hydrogels composed of alginate reinforced with hyaluronic acid may be an exquisite candidate for AKI intervention [ 75 ].
Chitosan Chitosan, a linear polysaccharide composed of randomly dispersed β- [ 1 , 2 , 3 , 4 ]-linked d -glucosamine (deacetylated unit) and N -acetyl- d -glucosamine (acetylated unit), derived from chitin, is the second most abundant natural biopolymer available on earth [ 70 ]. Chitosan can be found in the exoskeleton of crustaceans and the cell envelope of fungi [ 60 ]. Aside from having structural similarity to glycosaminoglycans contained in the ECM, chitosan owns the features of biocompatibility, biodegradability, microbial resistance, non-toxicity and low cost [ 76 ]. This type of hydrogel is also sensitive to pH and temperature [ 65 ], which make it amenable to modification and be used in different kinds of conditions. Recent in vivo studies have highlighted the potency of chitosan-based hydrogels in improving the retention and survival as well as the therapeutic benefits of MSCs in AKI [ 39 , 42 ]. Though seemingly quite promising, the poor mechanical strength and water solubility of chitosan under physiological conditions limit its use in biomedical applications. Conjugation with peptides or other hydrogels may assist to overcome some of these drawbacks [ 48 , 65 ].
Hyaluronic acid Hyaluronic acid (HA) is a relatively high molecular weight non-sulfated glycosaminoglycan (GAG) containing repeated units of (β-1,4)-linked d -glucuronic acid and (β-1,3)-linked N -acetyl- d -glucosamine [ 77 ]. It is widely distributed throughout the body of adult mammals including connective tissue, synovial fluid, and vitreous humor [ 61 , 78 ]. HA is an important component of ECM and is essential for cell growth, angiogenesis, embryonic development, wound healing, matrix organization, and morphogenesis [ 79 ]. HA has some remarkable properties such as non-adhesiveness, native bio-functionality, hydrophilia, and biodegradability [ 61 , 65 ]. These advantages make hydrogels built from HA increasingly versatile for a myriad of biomedical applications. Evidence has shown that HA hydrogels can facilitate cell migration and adhesion by binding to the transmembrane receptor CD44 [ 80 ]. The implantation of stem cells into the HA hydrogels affects the release of cytokines/chemokines, counterbalancing the secretion of proinflammatory mediators from the immune cells, thereby influencing the immune response and ameliorating the renal damage [ 81 ]. Moreover, the highly reversible thermal properties of the HA hydrogels offer great conditions for their use as an injectable scaffold for the culture and delivery of MSCs or an implant material for the repair and reconstruction of the soft and hard tissues [ 82 ]. However, for the lack of stability at the body temperature and the ability of controlled release of the bioactive molecules, necessary methods such as chemical modification and covalent crosslink are needed to improve the performance of HA hydrogels [ 83 ].
Collagen Collagen, a fiber-like structure, is the most abundant structural protein present in the mammalian ECM [ 62 ]. Collagen exhibits a unique triple-helical structure with a repeating amino acid sequence (Gly-X–Y)n [ 84 ]. It can be easily manipulated through chemical and physical cross-linking or by blending with other polymers [ 85 ]. Owing to its biocompatibility, biodegradability, elasticity as well as structural similarity to the tissues, collagen hydrogels have been frequently investigated as a biomimetic 3D culture scaffold to support cell growth [ 70 ]. Previous studies have demonstrated that collagen-based scaffolds can enhance cell retention, cell functionality, cell proliferation, and phenotype maintenance, which thereby increases the therapeutic effects of MSCs for AKI [ 31 , 37 , 70 ]. Nevertheless, pure collagen scaffolds have weak structural stability and mechanical strength [ 86 ]. Recent advances in scaffold formulation have contributed to the improvement in the collagen-based hydrogel system.
Matrigel Matrigel, a basement–membrane matrix generated from Engelbreth–Holm–Swarm (EHS) mouse sarcomas, is a widely used collagen-containing hydrogel in tissue engineering applications [ 87 ]. The primary components of Matrigel are laminin, collagen IV, entactin, and the heparin sulfate proteoglycan [ 88 ]. Matrigel also contains a series of growth factors such as transforming growth factor (TGF) family peptides, fibroblast growth factors (FGFs), and insulin-like growth factors (IGFs), as well as enzymes [ 89 ]. Collectively, these components contribute to the excellent biological function of Matrigel. Although Matrigel has been tested as a cell culture tool for several decades [ 88 ], its applicability is severely limited due to its ill-defined, complex, and variable constituent [ 90 ]. The undefined compositions and antigenicity of Matrigel may lead to batch-to-batch differences in mechanical and biochemical characteristics in cell culture experiments, making it hard to characterize cell behavior and reproduce, which are major hurdles in fundamental research [ 87 ].
ECM Kidney ECM hydrogels, obtained through decellularization process, have attracted substantial attention in recent years as new solutions to kidney injuries [ 91 ]. By removing the cellular components and retaining the proteins, glycosaminoglycans, as well as growth factors present in the native tissue, these hydrogels are nonimmunogenic, biocompatible, and biologically active [ 92 , 93 ]. Notably, in contrast to hydrogels composed of individual ECM components, kidney ECM hydrogels reserve the full biochemical complexity of the kidney tissue and, unlike Matrigel, do not consist of proteins originated from tumorigenic cells [ 94 ]. Kidney ECM hydrogels are currently being evaluated as an injectable scaffold to facilitate the repair and reconstruction of the renal tissue, and the results are encouraging [ 91 , 95 ]. Nevertheless, like all natural materials, the properties of kidney ECM hydrogels and the effects of these properties upon cell behaviors are neither well understood nor controlled [ 94 ]. Future studies should further elucidate these issues, providing further insight into the management of AKI.
Gelatin As a hydrolytic product of natural collagen, typically of bovine or porcine origin, gelatin is a biocompatible and biodegradable polypeptide containing 18 different kinds of amino acids [ 70 , 96 ]. In contrast to collagen, gelatin does not elicit any noticeable antigenicity under physiological conditions [ 96 ]. In addition to the above advantages, gelatin also has some other desirable properties such as commercial availability, cost economy, water solubility, adhesiveness, and easy processability, making it attractive in the applications of biomedicine [ 63 ]. MSCs-laden gelatin-based hydrogels have been shown to prolong the survival of MSCs and thus promote the repair of injured tissues in experimental AKI models [ 32 ]. Still, there are disadvantages existed in gelatin hydrogels, including poor mechanical strength, rapid enzymatic degradation, and inferior heat stability [ 97 ]. It is well known that pure gelatin has a solgel transition point around body temperature [ 98 ]. Therefore, pure gelatin could be injected as a low-viscosity fluid at 37 °C, but failed to form a stable hydrogel in vivo. Further modification is required to help improve the overall properties of native gelatin.
Fibrin Fibrin is a kind of natural polymer derived from key proteins involved in the blood clotting process [ 64 ]. In other words, it comes from fibrinogen and thrombin. The morphology, mechanical properties, and stability of fibrin hydrogels can be easily modulated by controlling the ratio of fibrinogen and thrombin in the hydrogels [ 99 ]. Fibrin-based hydrogels have been widely utilized for culturing and delivering MSCs due to their unique viscoelastic behavior, biocompatibility, biodegradability, and hemostasis [ 100 , 101 , 102 ]. When used as cell delivery systems, fibrin hydrogels have the advantage of being able to be implanted through injection without invasive surgery [ 51 ]. However, fibrin hydrogels face the challenges of weak mechanical strength and fast degradation speed, which limit their applications in renal diseases [ 48 ]. Researchers have tried to combine fibrin with other molecules or biomaterials to enhance its inherent biological properties. The usability and validity of these fibrin-based hydrogels in AKI have yet to be clarified.
Synthetic polymer hydrogels
Synthetic hydrogels are constructed with industrially manufactured polymers. Unlike natural hydrogels, synthetic hydrogels provide researchers with highly versatile materials that can be precisely controlled and designed [ 103 , 104 ]. In addition, many synthetic hydrogels are essentially bioinert, allowing engineers to specifically modulate the cell–material interactions [ 77 ]. In this perspective, more predictable results can be achieved. Poly (ethylene glycol) (PEG) is the most widely implemented bioinert synthetic polymer in 3D cell culture [ 103 ]. Other commonly used synthetic hydrogels include poly (vinyl alcohol) (PVA) and poly [ 2 -hydroxyethyl methacrylate] (PHEMA) [ 103 ].
Poly (ethylene glycol) (PEG) Poly (ethylene glycol) (PEG), sometimes referred to as poly (ethylene oxide) (PEO) depending on its molecular weight, is a very popular synthetic hydrophilic polymer used for hydrogel formation [ 85 ]. The basic structure of PEG is PEG diol with hydroxyl groups at each terminus, which can be converted into other functional groups like methyloxyl, carboxyl, amine, thiol, azide, vinyl sulfone, acetylene, and acrylate [ 105 ]. PEG has been considered as an ideal candidate for cell culture due to its non-toxicity to living tissues, superior resistance to protein adsorption, and ease of modification [ 106 ]. Its relatively low protein absorption prevents undesired cell–matrix reactions on the one hand, while, on the other hand, it also precludes this kind of material from having any interactions with the cells, which plays an important instructive role in mediating cell growth and functions [ 107 ]. Consequently, this type of hydrogel needs to be further modified with peptides or proteins, allowing individual control over each property of the matrix. Although recent work has shown that PEG-based hydrogels could increase stem cell attachment and proliferation [ 108 , 109 , 110 ], few are applied in the treatment of AKI. Thus, additional studies are required to further evaluate their effect on the kidneys.
Poly (vinyl alcohol) (PVA) Poly (vinyl alcohol) (PVA) is a water-soluble semicrystalline synthetic polymer with a backbone composed only of carbon atoms [ 111 ]. PVA is also a type of protein-resistant hydrogel and offers great flexibility in terms of precursor design [ 112 ]. PVA has received great attention in biomedical fields because of its advantages such as biodegradability, non-toxicity, non-carcinogenicity, and excellent mechanical properties [ 113 , 114 , 115 ]. Although some previous studies have indicated the facilitating effect of PVA on MSCs proliferation and its safety for in vivo use [ 111 , 116 , 117 ], evidence is still lacking in renal application.
Poly [2-hydroxyethyl methacrylate] (PHEMA) Poly [2-hydroxyethyl methacrylate] (PHEMA), one of the most important members of the methacrylate polymers, is the first successfully employed hydrogel in biological fields [ 118 ]. The presence of free hydroxyl group in PHEMA leads to the highly hydrophilic nature of this hydrogel, which facilitates the transportation of solutes and oxygen [ 119 , 120 ]. This property in conjunction with other properties like cytocompatibility, non-toxicity, and ease of tuning makes hydrogel fabricated from PHEMA a fit candidate for biomedical use, especially for controlled drug release [ 120 , 121 , 122 ]. However, PHEMA is relatively weak in mechanical strength and is considered nonbiodegradable, limiting its application in vivo. In this regard, modifications have to be made by incorporating some enzymatically susceptible monomers or cross-linking agents into the PHEMA hydrogels [ 103 ]. Similar to PEG and PVA, although modified PHEMA hydrogels have been shown to promote the attachment, spread, and proliferation of MSCs, their effects on the kidney have not been validated yet [ 123 , 124 ].
Hybrid hydrogels
Both natural and synthetic hydrogels have their own advantages and disadvantages. To overcome the inherent drawbacks of the traditional single-component hydrogels, researchers have been devoting efforts to combine multiple kinds of polymers to form hybrid hydrogels [ 125 ]. In this regard, hydrogels can be endowed with some particularly desirable characteristics to better mimic the native microenvironment. Generally, these combinations can be classified into three types: [1] a mixture of two or more ingredients that can form hydrogels alone; [2] a cocktail of the materials in which at least one of them cannot form hydrogels alone; and [3] a mix of the functional groups and the biomaterials. Recent studies [ 32 , 34 , 37 , 39 , 42 , 81 ] have reported that hybrid hydrogels displayed wonderful potency in enhancing the engraftment as well as the survival of MSCs, thereby accelerating the renal functional recovery (Table 2 ). However, as the use of these hydrogels for AKI is relatively new and there is only a modest amount of data about their performance on the kidneys, further research regarding their in vivo efficacy and safety is still needed.
Spheroid culture
Spheroid culture is also a promising method for 3D cell culture. Through this method, 3D aggregations of MSCs and their secreted ECM could be obtained without the involvement of a scaffold mimicking the real tissues. Several techniques have been used for spheroid fabrication, including hanging drop, liquid overlay, spinner culture, pellet culture, and magnetic levitation [ 126 , 127 ].
The hanging drop technique was the earliest described method used for spheroid fabrication [ 127 ]. In this method, cells gather at the bottom of the droplet and spontaneously aggregate to form spheroids. Hanging drop culture has many advantages such as controllable spheroid size and no need for professional equipment [ 126 ]. Alternatively, liquid overlay also enables cell aggregation and is suitable for large-scale production. It allows cells to grow in plates with substrates that limit cell adhesion. Typically, the non-adherent substrate is composed of agarose or PEG [ 128 ]. Another popular method for spheroid formation is spinner culture. In this system, cell suspension is put into a flask which is continuously stirred. This approach is especially amenable for long-term culture and intensive cell expansion in addition to mass production [ 129 ]. Spheroids can also be generated by centrifugation, which is often referred to as the method of pellet culture. This method is commonly used to induce the differentiation of MSCs [ 126 ]. A more recently developed technique for spheroid culture is magnetic levitation. The resultant spheroids can be easily manipulated and tracked via this means [ 130 ]. In general, with the help of 3D spheroid culture, MSCs could better maintain their distinct phenotypic and functional properties as well as secrete higher levels of cytokines or other factors, which as a result, improves the therapeutic effects of MSCs for AKI [ 131 , 132 ].
Preconditioning methods
Another critical bottleneck in the field of MSCs therapy is the harsh endogenous environment where the cells are located after transplantation [ 133 ]. This chokepoint has sparked the creation of preconditioning strategy (Fig. 3 ). Currently, researchers have attempted to pretreat MSCs with various physical, chemical, or biological factors to improve their efficacy in preclinical AKI models and the results are promising (Table 3 ).
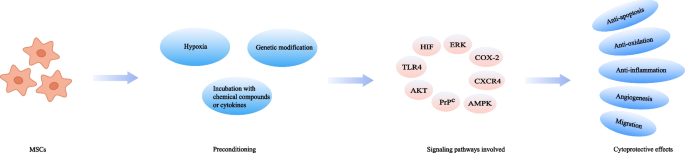
Preconditioning strategies for MSCs-based therapy in AKI models
Hypoxia preconditioning has been frequently applied to improve the therapeutic potential of MSCs. MSCs are cultured in the environment with a 21% oxygen level generally, but once transplanted into the injured tissues, they often encounter hypoxic conditions with oxygen concentrations ranging from 1 to 6% [ 134 ]. The changed culture oxygen tension could affect a wide variety of cellular activities, including proliferation, differentiation, senescence, and metabolism, which may consequently compromise the cell ability for repairing dysfunctional organs [ 135 ]. Pre-exposure of MSCs to hypoxia could help conquer this obstacle. As reported in previous studies, hypoxic pretreated MSCs remarkably accelerate the functional and histological recovery in ischemic AKI models [ 40 , 136 , 137 ], which hypoxia-inducible factor-1α (HIF-1α) is thought to play a crucial role in this process [ 138 ].
Incubation with chemical compounds or cytokines
Pre-incubation of MSCs with various chemical compounds or cytokines has also been proved as an effective tool to improve the therapeutic efficacy of MSCs. Currently documented chemicals and biomacromolecules used for MSCs pretreatment in preclinical AKI models include melatonin, atorvastatin, and insulin growth factor-1 (IGF-1) (Table 3 ).
Melatonin is a neurohormone secreted by the pineal gland, having a variety of functions such as circadian rhythms regulation, anti-inflammation, and anti-oxidation [ 139 , 140 ]. It was documented that MSCs pretreated with melatonin led to an enhanced therapeutic outcome in AKI models. The underlying mechanism might be that melatonin could suppress reactive oxygen species (ROS) generation and oxidative stress in either a receptor-dependent manner through ERK1/2, AMPK/ACC, and PrP C /PINK1 signaling pathways, or receptor-independent manner [ 141 , 142 ]. The HMG-CoA reductase inhibitor atorvastatin has also been tested for treating AKI because of its anti-apoptotic, antioxidant, and anti-inflammatory effects [ 143 ]. Incubation of MSCs with atorvastatin prior to transplantation increased the viability of MSCs, resulting in the promotion of renal recovery. HMGB1/TLR4 pathway is considered to play a pivotal role during this process [ 144 ]. Another potential cell protective reagent is S‐nitroso N‐acetyl penicillamine (SNAP). It is a nitric oxide (NO) donor with the ability to regulate hemodynamics. In a model of renal ischemia/reperfusion (I/R) injury, MSCs preconditioned with SNAP were found more effective than those untreated, which was accompanied by an increase in the expression of PI3K/AKT pathway-related proteins [ 145 ]. 14S,21Rdihydroxy-docosa4Z,7Z,10Z,12E,16Z,19Z-hexaenoic acid (14S,21R-diHDHA) is also a cytoprotective agent exerting its beneficial effects on MSCs via PI3K/AKT pathway. A study demonstrated that preconditioning of MSCs with 14S,21R-diHDHA was able to ameliorate renal dysfunction and renal histological injury [ 146 ]. Similarly, the administration of MSCs primed with hyaluronan monoesters with butyric acid (HB), a differentiating agent, helped decrease the level of inflammation, which consequently reinforced the effectiveness of MSCs-based treatment in ischemic AKI [ 43 ].
The interaction between cytokines and their receptors can activate signaling cascades relevant to cell survival, proliferation, and migration. Therefore, cytokines preconditioning may have an impact on the fate of MSCs in vitro and in vivo. Interleukin-17A (IL-17A) pretreatment protected MSCs from harmful immune response, which thereby consolidated the therapeutic utility of MSCs. This improved effect was proved to be due to the increase in Treg percentages through the COX-2/PGE2 pathway [ 147 ]. Likewise, preconditioning with IGF-1 enhanced the migration of MSCs, leading to an improvement in the therapeutically relevant effects. An overexpression of CXCR4 was observed in this preconditioning method, which was considered to be associated with the increased migratory capacity [ 148 ].
Genetic modification
Another approach employed to increase the therapeutic potency of MSCs is genetic manipulation. Recent data have indicated that several genes are related to the function of MSCs and thus could be targets for modification. For example, heme oxygenase-1 (HO-1) is highly correlated with anti-oxidative activity and vascular endothelial growth factor (VEGF) is responsible for angiogenesis [ 149 ]. By overexpressing these specific factors, the migration ability, vasculotropic action, as well as the anti-inflammatory and survival capacities of MSCs could be boosted, contributing to the better recovery of renal function. PI3K/Akt, MEK/ERK, and other signaling pathways are involved in this cytoprotective process [ 150 , 151 , 152 , 153 , 154 , 155 , 156 , 157 , 158 , 159 , 160 , 161 , 162 , 163 ]. Furthermore, it should also be mentioned that in clinical practice, the application of genetic modification needs to be more prudent as consistent activation of some specific genes might be a risk factor for stem cell-derived tumors.
Conclusion and future perspective
In conclusion, MSCs hold a considerable promise for the treatment of AKI. Nevertheless, the major outcomes of MSCs therapy in clinical trials of AKI have fallen far short of the theoretical effects of MSCs in preclinical studies. Challenges remain with respect to the clinical translation of this stem cell-based therapy. To address these challenges, various regimens including local administration, 3D cell culture as well as preconditioning have been exploited. In addition, considering the heterogeneity among patients, it is also important to realize that “one-size-fits-all” approach is clinically outdated. The characteristics of the patients such as age, genetics, and overall health status should be taken into consideration when applying the aforementioned strategies. Further research focused on the optimization of MSCs-based therapy is still needed to achieve the maximum therapeutic efficiency of MSCs in AKI patients.
Availability of data and materials
Not applicable.
Abbreviations
- Acute kidney injury
Chronic kidney disease
Extracellular matrix
End-stage kidney disease
Hyaluronic acid
Hyaluronan monoesters with butyric acid
Heme oxygenase-1
Intra-arterial
Insulin growth factor-1
Interleukin-17A
Ischemia/reperfusion
Intravenous
- Mesenchymal stem cells
Microvesicles
Nitric oxide
Poly(ethylene glycol)
Poly(2-hydroxyethyl methacrylate)
Poly(vinyl alcohol)
Reactive oxygen species
Renal replacement therapy
S‐nitroso N ‐acetyl penicillamine
Vascular endothelial growth factor
14S,21Rdihydroxy-docosa4Z,7Z,10Z,12E,16Z,19Z-hexaenoic acid
Two-dimensional
Three-dimensional
Ronco C, Bellomo R, Kellum JA. Acute kidney injury. Lancet. 2019;394(10212):1949–64.
Article CAS PubMed Google Scholar
Lameire NH, Bagga A, Cruz D, De Maeseneer J, Endre Z, Kellum JA, et al. Acute kidney injury: an increasing global concern. Lancet. 2013;382(9887):170–9.
Article PubMed Google Scholar
Mehta RL, Cerda J, Burdmann EA, Tonelli M, Garcia-Garcia G, Jha V, et al. International Society of Nephrology’s 0by25 initiative for acute kidney injury (zero preventable deaths by 2025): a human rights case for nephrology. Lancet. 2015;385(9987):2616–43.
Sato Y, Takahashi M, Yanagita M. Pathophysiology of AKI to CKD progression. Semin Nephrol. 2020;40(2):206–15.
Chawla LS, Eggers PW, Star RA, Kimmel PL. Acute kidney injury and chronic kidney disease as interconnected syndromes. N Engl J Med. 2014;371(1):58–66.
Article PubMed PubMed Central Google Scholar
See EJ, Jayasinghe K, Glassford N, Bailey M, Johnson DW, Polkinghorne KR, et al. Long-term risk of adverse outcomes after acute kidney injury: a systematic review and meta-analysis of cohort studies using consensus definitions of exposure. Kidney Int. 2019;95(1):160–72.
Rewa O, Bagshaw SM. Acute kidney injury-epidemiology, outcomes and economics. Nat Rev Nephrol. 2014;10(4):193–207.
Thakar CV. Perioperative acute kidney injury. Adv Chronic Kidney Dis. 2013;20(1):67–75.
Moore PK, Hsu RK, Liu KD. Management of acute kidney injury: core curriculum 2018. Am J Kidney Dis. 2018;72(1):136–48.
Kou M, Huang L, Yang J, Chiang Z, Chen S, Liu J, et al. Mesenchymal stem cell-derived extracellular vesicles for immunomodulation and regeneration: a next generation therapeutic tool? Cell Death Dis. 2022;13(7):580.
Article CAS PubMed PubMed Central Google Scholar
Khubutiya MS, Vagabov AV, Temnov AA, Sklifas AN. Paracrine mechanisms of proliferative, anti-apoptotic and anti-inflammatory effects of mesenchymal stromal cells in models of acute organ injury. Cytotherapy. 2014;16(5):579–85.
de Almeida DC, Donizetti-Oliveira C, Barbosa-Costa P, Origassa CS, Camara NO. In search of mechanisms associated with mesenchymal stem cell-based therapies for acute kidney injury. Clin Biochem Rev. 2013;34(3):131–44.
PubMed PubMed Central Google Scholar
Liang XT, Ding Y, Zhang YL, Tse HF, Lian QZ. Paracrine mechanisms of mesenchymal stem cell-based therapy: current status and perspectives. Cell Transplant. 2014;23(9):1045–59.
Pittenger MF, Discher DE, Peault BM, Phinney DG, Hare JM, Caplan AI. Mesenchymal stem cell perspective: cell biology to clinical progress. NPJ Regen Med. 2019;4:22.
Fan M, Zhang J, Xin H, He X, Zhang X. Current perspectives on role of MSC in renal pathophysiology. Front Physiol. 2018;9:1323.
Morigi M, Imberti B, Zoja C, Corna D, Tomasoni S, Abbate M, et al. Mesenchymal stem cells are renotropic, helping to repair the kidney and improve function in acute renal failure. J Am Soc Nephrol. 2004;15(7):1794–804.
Lange C, Togel F, Ittrich H, Clayton F, Nolte-Ernsting C, Zander AR, et al. Administered mesenchymal stem cells enhance recovery from ischemia/reperfusion-induced acute renal failure in rats. Kidney Int. 2005;68(4):1613–7.
Bruno S, Grange C, Deregibus MC, Calogero RA, Saviozzi S, Collino F, et al. Mesenchymal stem cell-derived microvesicles protect against acute tubular injury. J Am Soc Nephrol. 2009;20(5):1053–67.
Swaminathan M, Stafford-Smith M, Chertow GM, Warnock DG, Paragamian V, Brenner RM, et al. Allogeneic mesenchymal stem cells for treatment of AKI after cardiac surgery. J Am Soc Nephrol. 2018;29(1):260–7.
Rodrigues CE, Capcha JM, de Braganca AC, Sanches TR, Gouveia PQ, de Oliveira PA, et al. Human umbilical cord-derived mesenchymal stromal cells protect against premature renal senescence resulting from oxidative stress in rats with acute kidney injury. Stem Cell Res Ther. 2017;8(1):19.
Wechsler ME, Rao VV, Borelli AN, Anseth KS. Engineering the MSC secretome: a hydrogel focused approach. Adv Healthc Mater. 2021;10(7):e2001948.
Sanchez-Diaz M, Quinones-Vico MI, Sanabria de la Torre R, Montero-Vilchez T, Sierra-Sanchez A, Molina-Leyva A, et al. Biodistribution of mesenchymal stromal cells after administration in animal models and humans: a systematic review. J Clin Med. 2021;10(13):2925.
Fischer UM, Harting MT, Jimenez F, Monzon-Posadas WO, Xue H, Savitz SI, et al. Pulmonary passage is a major obstacle for intravenous stem cell delivery: the pulmonary first-pass effect. Stem Cells Dev. 2009;18(5):683–92.
Schrepfer S, Deuse T, Reichenspurner H, Fischbein MP, Robbins RC, Pelletier MP. Stem cell transplantation: the lung barrier. Transpl Proc. 2007;39(2):573–6.
Article CAS Google Scholar
Schmuck EG, Koch JM, Centanni JM, Hacker TA, Braun RK, Eldridge M, et al. Biodistribution and clearance of human mesenchymal stem cells by quantitative three-dimensional cryo-imaging after intravenous infusion in a rat lung injury model. Stem Cells Transl Med. 2016;5(12):1668–75.
Kyriakou C, Rabin N, Pizzey A, Nathwani A, Yong K. Factors that influence short-term homing of human bone marrow-derived mesenchymal stem cells in a xenogeneic animal model. Haematologica. 2008;93(10):1457–65.
Cui LL, Kerkela E, Bakreen A, Nitzsche F, Andrzejewska A, Nowakowski A, et al. The cerebral embolism evoked by intra-arterial delivery of allogeneic bone marrow mesenchymal stem cells in rats is related to cell dose and infusion velocity. Stem Cell Res Ther. 2015;6:11.
Lalu MM, McIntyre L, Pugliese C, Fergusson D, Winston BW, Marshall JC, et al. Safety of cell therapy with mesenchymal stromal cells (SafeCell): a systematic review and meta-analysis of clinical trials. PLoS ONE. 2012;7(10):e47559.
Kansu E. Thrombosis in stem cell transplantation. Hematology. 2012;17(Suppl 1):S159–62.
Bagno LL, Salerno AG, Balkan W, Hare JM. Mechanism of action of mesenchymal stem cells (MSCs): impact of delivery method. Expert Opin Biol Ther. 2022;22(4):449–63.
Huang M, Li D, Chen J, Ji Y, Su T, Chen Y, et al. Comparison of the treatment efficacy of umbilical mesenchymal stem cell transplantation via renal subcapsular and parenchymal routes in AKI-CKD mice. Stem Cell Res Ther. 2022;13(1):128.
Fu Z, Chu Y, Geng X, Ma Y, Chi K, Song C, et al. Artificial kidney capsule packed with mesenchymal stem cell-laden hydrogel for the treatment of rhabdomyolysis-induced acute kidney injury. ACS Biomater Sci Eng. 2022;8(4):1726–34.
Yang YJ, Geng XD, Chi K, Liu C, Liu R, Chen XM, et al. Ultrasound enhances the therapeutic potential of mesenchymal stem cells wrapped in greater omentum for aristolochic acid nephropathy. Stem Cell Res Therapy. 2021;12(1):261.
Wang H, Shang Y, Chen X, Wang Z, Zhu D, Liu Y, et al. Delivery of MSCs with a hybrid beta-sheet peptide hydrogel consisting IGF-1C domain and D-form peptide for acute kidney injury therapy. Int J Nanomed. 2020;15:4311–24.
Paglione D, Gatta L, Cavallari G, Perri A, Bonofiglio R, Catalano S, et al. Acute kidney ischemic injury in a rat model treated by human omental mesenchymal stem cells. Transpl Proc. 2020;52(10):2977–9.
Havakhah S, Sankian M, Kazemzadeh GH, Sadri K, Bidkhori HR, Naderi-Meshkin H, et al. In vivo effects of allogeneic mesenchymal stem cells in a rat model of acute ischemic kidney injury. Iran J Basic Med Sci. 2018;21(8):824–31.
Huang S, Li Y, Wang X, Ma X, Zhang X. Injectable co-gels of collagen and decellularized vascular matrix improve MSC-based therapy for acute kidney injury. J Biomater Sci Polym Ed. 2017;28(18):2186–95.
Geng X, Hong Q, Wang W, Zheng W, Li O, Cai G, et al. Biological membrane-packed mesenchymal stem cells treat acute kidney disease by ameliorating mitochondrial-related apoptosis. Sci Rep. 2017;7:41136.
Feng G, Zhang J, Li Y, Nie Y, Zhu D, Wang R, et al. IGF-1 C domain-modified hydrogel enhances cell therapy for AKI. J Am Soc Nephrol. 2016;27(8):2357–69.
Zhang W, Liu L, Huo Y, Yang Y, Wang Y. Hypoxia-pretreated human MSCs attenuate acute kidney injury through enhanced angiogenic and antioxidative capacities. Biomed Res Int. 2014;2014:462472.
Cheng K, Rai P, Plagov A, Lan X, Kumar D, Salhan D, et al. Transplantation of bone marrow-derived MSCs improves cisplatinum-induced renal injury through paracrine mechanisms. Exp Mol Pathol. 2013;94(3):466–73.
Gao J, Liu R, Wu J, Liu Z, Li J, Zhou J, et al. The use of chitosan based hydrogel for enhancing the therapeutic benefits of adipose-derived MSCs for acute kidney injury. Biomaterials. 2012;33(14):3673–81.
La Manna G, Bianchi F, Cappuccilli M, Cenacchi G, Tarantino L, Pasquinelli G, et al. Mesenchymal stem cells in renal function recovery after acute kidney injury: use of a differentiating agent in a rat model. Cell Transplant. 2011;20(8):1193–208.
Yang WY, Chen LC, Jhuang YT, Lin YJ, Hung PY, Ko YC, et al. Injection of hybrid 3D spheroids composed of podocytes, mesenchymal stem cells, and vascular endothelial cells into the renal cortex improves kidney function and replenishes glomerular podocytes. Bioeng Transl Med. 2021;6(2):e10212.
Engler AJ, Sen S, Sweeney HL, Discher DE. Matrix elasticity directs stem cell lineage specification. Cell. 2006;126(4):677–89.
Tietze S, Krater M, Jacobi A, Taubenberger A, Herbig M, Wehner R, et al. Spheroid Culture of Mesenchymal Stromal Cells Results in Morphorheological Properties Appropriate for Improved Microcirculation. Advanced science (Weinheim, Baden-Wurttemberg, Germany). 2019;6(8):1802104.
Wong KU, Zhang A, Akhavan B, Bilek MM, Yeo GC. Biomimetic culture strategies for the clinical expansion of mesenchymal stromal cells. ACS Biomater Sci Eng. 2021.
Hu X, Xia Z, Cai K. Recent advances in 3D hydrogel culture systems for mesenchymal stem cell-based therapy and cell behavior regulation. J Mater Chem B. 2022;10(10):1486–507.
Zhao Y, Song S, Wang D, Liu H, Zhang J, Li Z, et al. Nanozyme-reinforced hydrogel as a H(2)O(2)-driven oxygenerator for enhancing prosthetic interface osseointegration in rheumatoid arthritis therapy. Nat Commun. 2022;13(1):6758.
Liu XY, Liu J, Lin ST, Zhao XH. Hydrogel machines. Mater Today. 2020;36:102–24.
Huang Q, Zou Y, Arno MC, Chen S, Wang T, Gao J, et al. Hydrogel scaffolds for differentiation of adipose-derived stem cells. Chem Soc Rev. 2017;46(20):6255–75.
Seliktar D. Designing cell-compatible hydrogels for biomedical applications. Science. 2012;336(6085):1124–8.
Vedadghavami A, Minooei F, Mohammadi MH, Khetani S, Rezaei Kolahchi A, Mashayekhan S, et al. Manufacturing of hydrogel biomaterials with controlled mechanical properties for tissue engineering applications. Acta Biomater. 2017;62:42–63.
Bai RB, Yang JW, Suo ZG. Fatigue of hydrogels. Eur J Mech a-Solid. 2019;74:337–70.
Article Google Scholar
Yap JX, Leo CP, Mohd Yasin NH, Show PL, Chu DT, Singh V, et al. Recent advances of natural biopolymeric culture scaffold: synthesis and modification. Bioengineered. 2022;13(2):2226–47.
Zhang C, Shang Y, Chen X, Midgley AC, Wang Z, Zhu D, et al. Supramolecular nanofibers containing arginine-glycine-aspartate (RGD) Peptides boost therapeutic efficacy of extracellular vesicles in kidney repair. ACS Nano. 2020;14(9):12133–47.
Jiang Y, Wang Y, Li Q, Yu C, Chu W. Natural polymer-based stimuli-responsive hydrogels. Curr Med Chem. 2020;27(16):2631–57.
Khodadadi Yazdi M, Taghizadeh A, Taghizadeh M, Stadler FJ, Farokhi M, Mottaghitalab F, et al. Agarose-based biomaterials for advanced drug delivery. J Control Release. 2020;326:523–43.
Zhang M, Zhao X. Alginate hydrogel dressings for advanced wound management. Int J Biol Macromol. 2020;162:1414–28.
Kou SG, Peters LM, Mucalo MR. Chitosan: a review of sources and preparation methods. Int J Biol Macromol. 2021;169:85–94.
Burdick JA, Prestwich GD. Hyaluronic acid hydrogels for biomedical applications. Adv Mater. 2011;23(12):H41-56.
Lee CH, Singla A, Lee Y. Biomedical applications of collagen. Int J Pharm. 2001;221(1–2):1–22.
Bello AB, Kim D, Kim D, Park H, Lee SH. Engineering and functionalization of gelatin biomaterials: from cell culture to medical applications. Tissue Eng Part B Rev. 2020;26(2):164–80.
Caliari SR, Burdick JA. A practical guide to hydrogels for cell culture. Nat Methods. 2016;13(5):405–14.
Wagenbrenner M, Mayer-Wagner S, Rudert M, Holzapfel BM, Weissenberger M. Combinations of hydrogels and mesenchymal stromal cells (MSCs) for cartilage tissue engineering—a review of the literature. Gels. 2021;7(4):217.
Firouzabadi H, Iranpoor N, Gholinejad M, Kazemi F. Agarose hydrogel as an effective bioorganic ligand and support for the stabilization of palladium nanoparticles. Application as a recyclable catalyst for Suzuki-Miyaura reaction in aqueous media. RSC Adv. 2011;1(6):1013–9.
Axpe E, Oyen ML. Applications of alginate-based bioinks in 3D bioprinting. Int J Mol Sci. 2016;17(12):1976.
Khatab S, Leijs MJ, van Buul G, Haeck J, Kops N, Nieboer M, et al. MSC encapsulation in alginate microcapsules prolongs survival after intra-articular injection, a longitudinal in vivo cell and bead integrity tracking study. Cell Biol Toxicol. 2020;36(6):553–70.
Ghanta RK, Aghlara-Fotovat S, Pugazenthi A, Ryan CT, Singh VP, Mathison M, et al. Immune-modulatory alginate protects mesenchymal stem cells for sustained delivery of reparative factors to ischemic myocardium. Biomater Sci. 2020;8(18):5061–70.
Troy E, Tilbury MA, Power AM, Wall JG. Nature-based biomaterials and their application in biomedicine. Polymers (Basel). 2021;13(19):3321.
Lee KY, Mooney DJ. Alginate: properties and biomedical applications. Prog Polym Sci. 2012;37(1):106–26.
Dhamecha D, Movsas R, Sano U, Menon JU. Applications of alginate microspheres in therapeutics delivery and cell culture: past, present and future. Int J Pharm. 2019;569:118627.
Mohammadi M, Luong JC, Rodriguez SM, Cao R, Wheeler AE, Lau H, et al. Controlled release of stem cell secretome attenuates inflammatory response against implanted biomaterials. Adv Healthc Mater. 2020;9(12):e1901874.
Bari E, Scocozza F, Perteghella S, Sorlini M, Auricchio F, Torre ML, et al. 3D bioprinted scaffolds containing mesenchymal stem/stromal lyosecretome: next generation controlled release device for bone regenerative medicine. Pharmaceutics. 2021;13(4):515.
Amirian J, Van TTT, Bae SH, Jung HI, Choi HJ, Cho HD, et al. Examination of In vitro and In vivo biocompatibility of alginate-hyaluronic acid microbeads As a promising method in cell delivery for kidney regeneration. Int J Biol Macromol. 2017;105(Pt 1):143–53.
Huang Y, Onyeri S, Siewe M, Moshfeghian A, Madihally SV. In vitro characterization of chitosan-gelatin scaffolds for tissue engineering. Biomaterials. 2005;26(36):7616–27.
Slaughter BV, Khurshid SS, Fisher OZ, Khademhosseini A, Peppas NA. Hydrogels in regenerative medicine. Adv Mater. 2009;21(32–33):3307–29.
Ho TC, Chang CC, Chan HP, Chung TW, Shu CW, Chuang KP, et al. Hydrogels: properties and applications in biomedicine. Molecules. 2022;27(9):2902.
Arifka M, Wilar G, Elamin KM, Wathoni N. Polymeric hydrogels as mesenchymal stem cell secretome delivery system in biomedical applications. Polymers (Basel). 2022;14(6):1218.
Bhattacharya D, Svechkarev D, Souchek JJ, Hill TK, Taylor MA, Natarajan A, et al. Impact of structurally modifying hyaluronic acid on CD44 interaction. J Mater Chem B. 2017;5(41):8183–92.
Zullo JA, Nadel EP, Rabadi MM, Baskind MJ, Rajdev MA, Demaree CM, et al. The secretome of hydrogel-coembedded endothelial progenitor cells and mesenchymal stem cells instructs macrophage polarization in endotoxemia. Stem Cells Transl Med. 2015;4(7):852–61.
Shoma Suresh K, Bhat S, Guru BR, Muttigi MS, Seetharam RN. A nanocomposite hydrogel delivery system for mesenchymal stromal cell secretome. Stem Cell Res Ther. 2020;11(1):205.
Xu X, Jha AK, Harrington DA, Farach-Carson MC, Jia X. Hyaluronic acid-based hydrogels: from a natural polysaccharide to complex networks. Soft Matter. 2012;8(12):3280–94.
Mayo KH. NMR and x-ray studies of collagen model peptides. Biopolymers. 1996;40(4):359–70.
Naahidi S, Jafari M, Logan M, Wang Y, Yuan Y, Bae H, et al. Biocompatibility of hydrogel-based scaffolds for tissue engineering applications. Biotechnol Adv. 2017;35(5):530–44.
Dong C, Lv Y. Application of collagen scaffold in tissue engineering: recent advances and new perspectives. Polymers (Basel). 2016;8(2):42.
Aisenbrey EA, Murphy WL. Synthetic alternatives to Matrigel. Nat Rev Mater. 2020;5(7):539–51.
Kleinman HK, Martin GR. Matrigel: basement membrane matrix with biological activity. Semin Cancer Biol. 2005;15(5):378–86.
Benton G, Arnaoutova I, George J, Kleinman HK, Koblinski J. Matrigel: from discovery and ECM mimicry to assays and models for cancer research. Adv Drug Deliv Rev. 2014;79–80:3–18.
Talbot NC, Caperna TJ. Proteome array identification of bioactive soluble proteins/peptides in Matrigel: relevance to stem cell responses. Cytotechnology. 2015;67(5):873–83.
Sobreiro-Almeida R, Quinteira R, Neves NM. Renal regeneration: the role of extracellular matrix and current ECM-based tissue engineered strategies. Adv Healthc Mater. 2021;10(14):e2100160.
Kim JW, Nam SA, Yi J, Kim JY, Lee JY, Park SY, et al. Kidney decellularized extracellular matrix enhanced the vascularization and maturation of human kidney organoids. Adv Sci (Weinheim, Baden-Wurttemberg, Germany). 2022;9(15):e2103526.
Google Scholar
Song JJ, Guyette JP, Gilpin SE, Gonzalez G, Vacanti JP, Ott HC. Regeneration and experimental orthotopic transplantation of a bioengineered kidney. Nat Med. 2013;19(5):646–51.
Saldin LT, Cramer MC, Velankar SS, White LJ, Badylak SF. Extracellular matrix hydrogels from decellularized tissues: structure and function. Acta Biomater. 2017;49:1–15.
Zhou C, Zhou L, Liu J, Xu L, Xu Z, Chen Z, et al. Kidney extracellular matrix hydrogel enhances therapeutic potential of adipose-derived mesenchymal stem cells for renal ischemia reperfusion injury. Acta Biomater. 2020;115:250–63.
Rohanizadeh R, Swain MV, Mason RS. Gelatin sponges (Gelfoam) as a scaffold for osteoblasts. J Mater Sci Mater Med. 2008;19(3):1173–82.
Helminger M, Wu B, Kollmann T, Benke D, Schwahn D, Pipich V, et al. Synthesis and characterization of gelatin-based magnetic hydrogels. Adv Funct Mater. 2014;24(21):3187–96.
Mushtaq F, Raza ZA, Batool SR, Zahid M, Onder OC, Rafique A, et al. Preparation, properties, and applications of gelatin-based hydrogels (GHs) in the environmental, technological, and biomedical sectors. Int J Biol Macromol. 2022;218:601–33.
Tan J, Li L, Wang H, Wei L, Gao X, Zeng Z, et al. Biofunctionalized fibrin gel co-embedded with BMSCs and VEGF for accelerating skin injury repair. Mater Sci Eng C Mater Biol Appl. 2021;121:111749.
Heher P, Muhleder S, Mittermayr R, Redl H, Slezak P. Fibrin-based delivery strategies for acute and chronic wound healing. Adv Drug Deliv Rev. 2018;129:134–47.
Goetzke R, Franzen J, Ostrowska A, Vogt M, Blaeser A, Klein G, et al. Does soft really matter? Differentiation of induced pluripotent stem cells into mesenchymal stromal cells is not influenced by soft hydrogels. Biomaterials. 2018;156:147–58.
Ricles LM, Hsieh PL, Dana N, Rybalko V, Kraynak C, Farrar RP, et al. Therapeutic assessment of mesenchymal stem cells delivered within a PEGylated fibrin gel following an ischemic injury. Biomaterials. 2016;102:9–19.
Unal AZ, West JL. Synthetic ECM: bioactive synthetic hydrogels for 3D tissue engineering. Bioconjug Chem. 2020;31(10):2253–71.
Kloxin AM, Kloxin CJ, Bowman CN, Anseth KS. Mechanical properties of cellularly responsive hydrogels and their experimental determination. Adv Mater. 2010;22(31):3484–94.
Peppas NA, Keys KB, Torres-Lugo M, Lowman AM. Poly(ethylene glycol)-containing hydrogels in drug delivery. J Control Release. 1999;62(1–2):81–7.
Zhu J. Bioactive modification of poly(ethylene glycol) hydrogels for tissue engineering. Biomaterials. 2010;31(17):4639–56.
Browning MB, Russell B, Rivera J, Hook M, Cosgriff-Hernandez EM. Bioactive hydrogels with enhanced initial and sustained cell interactions. Biomacromol. 2013;14(7):2225–33.
Guerra AD, Rose WE, Hematti P, Kao WJ. Minocycline enhances the mesenchymal stromal/stem cell pro-healing phenotype in triple antimicrobial-loaded hydrogels. Acta Biomater. 2017;51:184–96.
Martin JR, Patil P, Yu F, Gupta MK, Duvall CL. Enhanced stem cell retention and antioxidative protection with injectable, ROS-degradable PEG hydrogels. Biomaterials. 2020;263:120377.
Xin S, Gregory CA, Alge DL. Interplay between degradability and integrin signaling on mesenchymal stem cell function within poly(ethylene glycol) based microporous annealed particle hydrogels. Acta Biomater. 2020;101:227–36.
Rivera-Hernandez G, Antunes-Ricardo M, Martinez-Morales P, Sanchez ML. Polyvinyl alcohol based-drug delivery systems for cancer treatment. Int J Pharm. 2021;600:120478.
Jiang S, Liu S, Feng W. PVA hydrogel properties for biomedical application. J Mech Behav Biomed Mater. 2011;4(7):1228–33.
Hassan CM, Peppas NA. Structure and applications of poly(vinyl alcohol) hydrogels produced by conventional crosslinking or by freezing/thawing methods. Adv Polym Sci. 2000;153:37–65.
Luo Q, Shan Y, Zuo X, Liu J. Anisotropic tough poly(vinyl alcohol)/graphene oxide nanocomposite hydrogels for potential biomedical applications. Rsc Adv. 2018;8(24):13284–91.
Rolim WR, Pieretti JC, Reno DLS, Lima BA, Nascimento MHM, Ambrosio FN, et al. Antimicrobial activity and cytotoxicity to tumor cells of nitric oxide donor and silver nanoparticles containing PVA/PEG films for topical applications. ACS Appl Mater Interfaces. 2019;11(6):6589–604.
Shafiee A, Soleimani M, Chamheidari GA, Seyedjafari E, Dodel M, Atashi A, et al. Electrospun nanofiber-based regeneration of cartilage enhanced by mesenchymal stem cells. J Biomed Mater Res A. 2011;99(3):467–78.
Gomide VS, Zonari A, Ocarino NM, Goes AM, Serakides R, Pereira MM. In vitro and in vivo osteogenic potential of bioactive glass-PVA hybrid scaffolds colonized by mesenchymal stem cells. Biomed Mater. 2012;7(1):015004.
Wichterle O, Lim D. Hydrophilic gels for biological use. Nature. 1960;185(4706):117–8.
Peng W, Lu X, Wu J, Wang Y, Zhu X, Ouyang H, et al. Autoclaving pHEMA-based hydrogels immersed in deionized water has no effect on physicochemical properties and cell behaviors. ACS Omega. 2022;7(36):32038–45.
Li J, Chee HL, Chong YT, Chan BQY, Xue K, Lim PC, et al. Hofmeister effect mediated strong PHEMA-gelatin hydrogel actuator. ACS Appl Mater Interfaces. 2022.
Lu SX, Anseth KS. Photopolymerization of multilaminated poly(HEMA) hydrogels for controlled release. J Control Release. 1999;57(3):291–300.
Noble ML, Mourad PD, Ratner BD. Digital drug delivery: on-off ultrasound controlled antibiotic release from coated matrices with negligible background leaching. Biomater Sci. 2014;2(6):839–902.
Kubinova S, Horak D, Sykova E. Cholesterol-modified superporous poly(2-hydroxyethyl methacrylate) scaffolds for tissue engineering. Biomaterials. 2009;30(27):4601–9.
Mohanty S, Alm M, Hemmingsen M, Dolatshahi-Pirouz A, Trifol J, Thomsen P, et al. 3D printed silicone-hydrogel scaffold with enhanced physicochemical properties. Biomacromol. 2016;17(4):1321–9.
Yang J, Zhang YS, Yue K, Khademhosseini A. Cell-laden hydrogels for osteochondral and cartilage tissue engineering. Acta Biomater. 2017;57:1–25.
Ryu NE, Lee SH, Park H. Spheroid culture system methods and applications for mesenchymal stem cells. Cells. 2019;8(12):1620.
Fennema E, Rivron N, Rouwkema J, van Blitterswijk C, de Boer J. Spheroid culture as a tool for creating 3D complex tissues. Trends Biotechnol. 2013;31(2):108–15.
Costa EC, de Melo-Diogo D, Moreira AF, Carvalho MP, Correia IJ. Spheroids formation on non-adhesive surfaces by liquid overlay technique: considerations and practical approaches. Biotechnol J. 2018;13(1).
Chaicharoenaudomrung N, Kunhorm P, Noisa P. Three-dimensional cell culture systems as an in vitro platform for cancer and stem cell modeling. World J Stem Cells. 2019;11(12):1065–83.
Lee SY, Lee JW. 3D Spheroid cultures of stem cells and exosome applications for cartilage repair. Life (Basel). 2022;12(7):939.
CAS PubMed Google Scholar
Cesarz Z, Tamama K. Spheroid culture of mesenchymal stem cells. Stem Cells Int. 2016;2016:9176357.
Xu Y, Shi T, Xu A, Zhang L. 3D spheroid culture enhances survival and therapeutic capacities of MSCs injected into ischemic kidney. J Cell Mol Med. 2016;20(7):1203–13.
Zhao L, Hu C, Zhang P, Jiang H, Chen J. Preconditioning strategies for improving the survival rate and paracrine ability of mesenchymal stem cells in acute kidney injury. J Cell Mol Med. 2019;23(2):720–30.
Stuart JA, Fonseca J, Moradi F, Cunningham C, Seliman B, Worsfold CR, et al. How Supraphysiological oxygen levels in standard cell culture affect oxygen-consuming reactions. Oxid Med Cell Longev. 2018;2018:8238459.
Buravkova LB, Andreeva ER, Gogvadze V, Zhivotovsky B. Mesenchymal stem cells and hypoxia: where are we? Mitochondrion. 2014;19(Pt A):105–12.
Yu X, Lu C, Liu H, Rao S, Cai J, Liu S, et al. Hypoxic preconditioning with cobalt of bone marrow mesenchymal stem cells improves cell migration and enhances therapy for treatment of ischemic acute kidney injury. PLoS ONE. 2013;8(5):e62703.
Liu H, Liu S, Li Y, Wang X, Xue W, Ge G, et al. The role of SDF-1-CXCR4/CXCR7 axis in the therapeutic effects of hypoxia-preconditioned mesenchymal stem cells for renal ischemia/reperfusion injury. PLoS ONE. 2012;7(4):e34608.
Silva LHA, Antunes MA, Dos Santos CC, Weiss DJ, Cruz FF, Rocco PRM. Strategies to improve the therapeutic effects of mesenchymal stromal cells in respiratory diseases. Stem Cell Res Ther. 2018;9(1):45.
Vasey C, McBride J, Penta K. Circadian rhythm dysregulation and restoration: the role of melatonin. Nutrients. 2021;13(10):3480.
Carrascal L, Nunez-Abades P, Ayala A, Cano M. Role of melatonin in the inflammatory process and its therapeutic potential. Curr Pharm Des. 2018;24(14):1563–88.
Chen HH, Lin KC, Wallace CG, Chen YT, Yang CC, Leu S, et al. Additional benefit of combined therapy with melatonin and apoptotic adipose-derived mesenchymal stem cell against sepsis-induced kidney injury. J Pineal Res. 2014;57(1):16–32.
Mias C, Trouche E, Seguelas MH, Calcagno F, Dignat-George F, Sabatier F, et al. Ex vivo pretreatment with melatonin improves survival, proangiogenic/mitogenic activity, and efficiency of mesenchymal stem cells injected into ischemic kidney. Stem cells (Dayton, Ohio). 2008;26(7):1749–57.
Yang YJ, Qian HY, Huang J, Geng YJ, Gao RL, Dou KF, et al. Atorvastatin treatment improves survival and effects of implanted mesenchymal stem cells in post-infarct swine hearts. Eur Heart J. 2008;29(12):1578–90.
Cai J, Yu X, Zhang B, Zhang H, Fang Y, Liu S, et al. Atorvastatin improves survival of implanted stem cells in a rat model of renal ischemia-reperfusion injury. Am J Nephrol. 2014;39(6):466–75.
Masoud MS, Anwar SS, Afzal MZ, Mehmood A, Khan SN, Riazuddin S. Pre-conditioned mesenchymal stem cells ameliorate renal ischemic injury in rats by augmented survival and engraftment. J Transl Med. 2012;10:243.
Tian H, Lu Y, Shah SP, Wang Q, Hong S. 14S,21R-dihydroxy-docosahexaenoic acid treatment enhances mesenchymal stem cell amelioration of renal ischemia/reperfusion injury. Stem Cells Dev. 2012;21(7):1187–99.
Bai M, Zhang L, Fu B, Bai J, Zhang Y, Cai G, et al. IL-17A improves the efficacy of mesenchymal stem cells in ischemic-reperfusion renal injury by increasing Treg percentages by the COX-2/PGE2 pathway. Kidney Int. 2018;93(4):814–25.
Xinaris C, Morigi M, Benedetti V, Imberti B, Fabricio AS, Squarcina E, et al. A novel strategy to enhance mesenchymal stem cell migration capacity and promote tissue repair in an injury specific fashion. Cell Transplant. 2013;22(3):423–36.
Xu H, Chen C, Hu L, Hou J. Gene-modified mesenchymal stem cell-based therapy in renal ischemia- reperfusion injury. Curr Gene Ther. 2017;17(6):453–60.
Ni W, Zhang Y, Yin Z. The protective mechanism of Klotho gene-modified bone marrow mesenchymal stem cells on acute kidney injury induced by rhabdomyolysis. Regen Ther. 2021;18:255–67.
Roudkenar MH, Halabian R, Tehrani HA, Amiri F, Jahanian-Najafabadi A, Roushandeh AM, et al. Lipocalin 2 enhances mesenchymal stem cell-based cell therapy in acute kidney injury rat model. Cytotechnology. 2018;70(1):103–17.
Yan X, Cheng X, He X, Zheng W, Yuan X, Chen H. HO-1 overexpressed mesenchymal stem cells ameliorate sepsis-associated acute kidney injury by activating JAK/stat3 pathway. Cell Mol Bioeng. 2018;11(6):509–18.
Liu N, Wang H, Han G, Cheng J, Hu W, Zhang J. Enhanced proliferation and differentiation of HO-1 gene-modified bone marrow-derived mesenchymal stem cells in the acute injured kidney. Int J Mol Med. 2018;42(2):946–56.
CAS PubMed PubMed Central Google Scholar
Mori da Cunha MG, Zia S, Beckmann DV, Carlon MS, Arcolino FO, Albersen M, et al. Vascular endothelial growth factor up-regulation in human amniotic fluid stem cell enhances nephroprotection after ischemia-reperfusion injury in the rat. Crit Care Med. 2017;45(1):e86–96.
Zhaleh F, Amiri F, Mohammadzadeh-Vardin M, Bahadori M, Harati MD, Roudkenar MH, et al. Nuclear factor erythroid-2 related factor 2 overexpressed mesenchymal stem cells transplantation, improves renal function, decreases injuries markers and increases repair markers in glycerol-induced Acute kidney injury rats. Iran J Basic Med Sci. 2016;19(3):323–9.
Liu N, Wang H, Han G, Tian J, Hu W, Zhang J. Alleviation of apoptosis of bone marrow-derived mesenchymal stem cells in the acute injured kidney by heme oxygenase-1 gene modification. Int J Biochem Cell Biol. 2015;69:85–94.
Mohammadzadeh-Vardin M, Habibi Roudkenar M, Jahanian-Najafabadi A. Adenovirus-mediated over-expression of Nrf2 within mesenchymal stem cells (MSCs) protected rats against acute kidney injury. Adv Pharm Bull. 2015;5(2):201–8.
Yuzeng Q, Weiyang H, Xin G, Qingson Z, Youlin K, Ke R. Effects of transplantation with marrow-derived mesenchymal stem cells modified with survivin on renal ischemia-reperfusion injury in mice. Yonsei Med J. 2014;55(4):1130–7.
Liu N, Patzak A, Zhang J. CXCR4-overexpressing bone marrow-derived mesenchymal stem cells improve repair of acute kidney injury. Am J Physiol Renal Physiol. 2013;305(7):F1064–73.
Yuan L, Wu MJ, Sun HY, Xiong J, Zhang Y, Liu CY, et al. VEGF-modified human embryonic mesenchymal stem cell implantation enhances protection against cisplatin-induced acute kidney injury. Am J Physiol Renal Physiol. 2011;300(1):F207–18.
Chen Y, Qian H, Zhu W, Zhang X, Yan Y, Ye S, et al. Hepatocyte growth factor modification promotes the amelioration effects of human umbilical cord mesenchymal stem cells on rat acute kidney injury. Stem Cells Dev. 2011;20(1):103–13.
Hagiwara M, Shen B, Chao L, Chao J. Kallikrein-modified mesenchymal stem cell implantation provides enhanced protection against acute ischemic kidney injury by inhibiting apoptosis and inflammation. Hum Gene Ther. 2008;19(8):807–19.
Togel F, Weiss K, Yang Y, Hu Z, Zhang P, Westenfelder C. Vasculotropic, paracrine actions of infused mesenchymal stem cells are important to the recovery from acute kidney injury. Am J Physiol Renal Physiol. 2007;292(5):F1626–35.
Download references
Acknowledgements
This work was supported by the National Key Research and Development Program of China (2018YFE0126600 and 2018YFA0108803), National Natural Science Foundation of China (No. 82020741, 82204744, 82270758 and 82170686), and the Fostering Fund of Chinese PLA General Hospital for National Distinguished Young Scholar Science Fund (2019-JQPY-002).
Author information
Zhangning Fu and Yifan Zhang contributed equally to this work
Authors and Affiliations
Medical School of Chinese PLA, Beijing, China
Zhangning Fu, Yifan Zhang & Kun Chi
Department of Nephrology, First Medical Center of Chinese PLA General Hospital, Nephrology Institute of the Chinese PLA, State Key Laboratory of Kidney Diseases, National Clinical Research Center for Kidney Diseases, Beijing Key Laboratory of Kidney Disease Research, Beijing, China
Zhangning Fu, Yifan Zhang, Xiaodong Geng, Kun Chi, Guangyan Cai, Xiangmei Chen & Quan Hong
Beidaihe Rehabilitation and Recuperation Center, Chinese People’s Liberation Army Joint Logistics Support Force, Qinhuangdao, China
Xiaodong Geng
Department of Critical Care Medicine, First Medical Center of Chinese PLA General Hospital, Beijing, China
Department of Nephrology, Beijing Electric Power Hospital, Beijing, China
Chengcheng Song
You can also search for this author in PubMed Google Scholar
Contributions
ZNF and QH designed the concept. ZNF, YFZ, XDG, KC, CL, and CCS searched the literature and collected the data. ZNF and YFZ drafted the manuscript. GYC, XMC, and QH revised the manuscript. All authors read and approved the final manuscript.
Corresponding author
Correspondence to Quan Hong .
Ethics declarations
Ethics approval and consent to participate, consent for publication, competing interests.
The authors declare that they have no competing interests.
Additional information
Publisher's note.
Springer Nature remains neutral with regard to jurisdictional claims in published maps and institutional affiliations.
Rights and permissions
Open Access This article is licensed under a Creative Commons Attribution 4.0 International License, which permits use, sharing, adaptation, distribution and reproduction in any medium or format, as long as you give appropriate credit to the original author(s) and the source, provide a link to the Creative Commons licence, and indicate if changes were made. The images or other third party material in this article are included in the article's Creative Commons licence, unless indicated otherwise in a credit line to the material. If material is not included in the article's Creative Commons licence and your intended use is not permitted by statutory regulation or exceeds the permitted use, you will need to obtain permission directly from the copyright holder. To view a copy of this licence, visit http://creativecommons.org/licenses/by/4.0/ . The Creative Commons Public Domain Dedication waiver ( http://creativecommons.org/publicdomain/zero/1.0/ ) applies to the data made available in this article, unless otherwise stated in a credit line to the data.
Reprints and permissions
About this article
Cite this article.
Fu, Z., Zhang, Y., Geng, X. et al. Optimization strategies of mesenchymal stem cell-based therapy for acute kidney injury. Stem Cell Res Ther 14 , 116 (2023). https://doi.org/10.1186/s13287-023-03351-2
Download citation
Received : 01 December 2022
Accepted : 20 April 2023
Published : 30 April 2023
DOI : https://doi.org/10.1186/s13287-023-03351-2
Share this article
Anyone you share the following link with will be able to read this content:
Sorry, a shareable link is not currently available for this article.
Provided by the Springer Nature SharedIt content-sharing initiative
- Local administration
- Three-dimensional culture
- Preconditioning
Stem Cell Research & Therapy
ISSN: 1757-6512
- Submission enquiries: Access here and click Contact Us
- General enquiries: [email protected]

An official website of the United States government
The .gov means it’s official. Federal government websites often end in .gov or .mil. Before sharing sensitive information, make sure you’re on a federal government site.
The site is secure. The https:// ensures that you are connecting to the official website and that any information you provide is encrypted and transmitted securely.
- Publications
- Account settings
Preview improvements coming to the PMC website in October 2024. Learn More or Try it out now .
- Advanced Search
- Journal List
- Medicine (Baltimore)
- v.102(21); 2023 May 26
- PMC10219694
Acute kidney injury in diabetic patients: A narrative review
Amninder kaur.
a Senior Resident, Department of Nephrology, All India Institute of Medical Sciences Rishikesh, Uttarakhand, India
Gaurav Shekhar Sharma
b Assistant Professor, Department of Nephrology, All India Institute of Medical Sciences Rishikesh, Uttrakhand, India
Damodar R Kumbala
c Diagnostic and Interventional Nephrologist, Renal Associates of Baton Rogue, Baton Rogue, LA.
Diabetes mellitus (DM) is the most common cause of chronic kidney disease, which leads to end-stage renal failure worldwide. Glomerular damage, renal arteriosclerosis, and atherosclerosis are the contributing factors in diabetic patients, leading to the progression of kidney damage. Diabetes is a distinct risk factor for acute kidney injury (AKI) and AKI is associated with faster advancement of renal disease in patients with diabetes. The long-term consequences of AKI include the development of end-stage renal disease, higher cardiovascular and cerebral events, poor quality of life, and high morbidity and mortality. In general, not many studies discussed extensively “AKI in DM.” Moreover, articles addressing this topic are scarce. It is also important to know the cause of AKI in diabetic patients so that timely intervention and preventive strategies can be implemented to decrease kidney injury. Aim of this review article is to address the epidemiology of AKI, its risk factors, different pathophysiological mechanisms, how AKI differs between diabetic and nondiabetic patients and its preventive and therapeutic implications in diabetics. The increasing occurrence and prevalence of AKI and DM, as well as other pertinent issues, motivated us to address this topic.
1. Introduction
Type 2 diabetes is one of the primary causes of chronic kidney disease (CKD) and end-stage renal disease (ESRD) globally. [ 1 ] Over the past 2 decades, diabetes mellitus incidence and prevalence have steadily increased, from an estimated 30 million in 1985 to 537 million in 2021 and it is anticipated that the number will increase to 643 million by 2030 and 783 million by 2045. [ 2 ] Considering recent trends, microvascular and macrovascular disorders are associated with long-term diabetes-related complications. One significant microvascular sequelae of diabetes are diabetic nephropathy (DN) or diabetic kidney disease (DKD). [ 3 ] Around 20% to 40% of diabetic patients are thought to suffer from DKD, and approximately 40% will need renal replacement therapy at some point in their lifetime. [ 4 , 5 ] Risk factors for the progression of CKD include gender, racial disparities, hereditary factors, concurrent comorbid illnesses, for example, diabetes mellitus (DM), metabolic abnormalities, and previous episodes of acute kidney injury (AKI), etc. [ 6 ]
AKI, which affects up to 1% of the general population and 15% of all hospitalized patients, is a worldwide health issue. [ 7 – 9 ] Diabetes is a distinct risk factor for AKI. [ 10 ] Although baseline DM is also an independent risk factor for AKI in multivariate analyses adjusted for estimated glomerular filtration rate (eGFR). [ 11 ] There have been other studies that showed that patients with diabetes may be more prone to AKI. [ 12 , 13 ] Acute tubular injury caused by renal insults may have an impact on kidney function, leading to chronic functional impairment and later maladaptive recovery and failure to entirely undo the insults. [ 14 , 15 ] Generally, there is a significant correlation between AKI and the emergence of CKD and ESRD. [ 16 , 17 ] In a cohort of 4082 patients with diabetes, Thakar et al [ 18 ] showed that AKI episodes were related to a cumulative likelihood of developing progressive CKD, regardless of the presence of any other significant risk factors for progression. Subsequently, a large prospective study provided additional evidence that AKI is a strong indicator of unfavorable outcomes (doubling of serum creatinine or ESRD) and mortality in diabetes. [ 19 ]
2. Epidemiology of AKI in diabetes mellitus
Girman et al [ 20 ] compared 119,966 diabetic patients with 1794,516 nondiabetic patients and showed that the incidence of AKI was significantly higher in diabetic patients (198 per 100,000 person-years vs 27 per 100,000 patient-years; crude hazard ratio, 8.0; 95% confidence interval, 7.4–8.7). Despite accounting for additional known comorbidities and AKI risk factors, the differences remained statistically significant. However, in their study, a clinical coding system rather than a biochemical definition of AKI was employed, which could result in significant under-ascertainment. Additionally, a meta-analysis by James et al revealed that participants with diabetes had higher AKI hazard ratios than participants without diabetes, regardless of their eGFR levels. Again, the AKI definition in these studies was based on administrative codes, which underestimated mild forms of AKI. [ 21 ]
In a retrospective cohort study by Prabhu et al [ 22 ] , an annual AKI incidence was 12.6%. There was a substantial deviation from previously published studies by Thakar et al [ 18 ] and Monseu et al [ 19 ] , who reported AKI incidence of 2.8% and 5.2%, respectively.
In their retrospective cohort of 16,700 participants (9417 with type 2 diabetes and 7283 nondiabetic controls), Hapca et al [ 23 ] found that diabetic patients had higher rates of AKI than controls (48.6% vs 17.2%, respectively). The AKI risk among diabetic patients was 5 times higher than that of controls, even in the absence of CKD (121.5 vs 24.6 per 1000 person-years). AKI rates in diabetic patients with CKD were twice as high as in controls (384.8 vs 180.0 per 1000 person-years after the onset of CKD, and 109.3 vs 47.4 per 1000 person-years before the onset of CKD).
Recently, Venot et al [ 24 ] in their prospective case-control study, which included patients with severe sepsis and septic shock with or without diabetes, found that the incidence of AKI did not differ between the 2 groups however, diabetic patients requiring dialysis more often, had higher mean serum creatinine levels, and less recovery than nondiabetic patients. However, this study has several limitations, as the diagnosis was made based on the medical history, long-term diabetic complications, and HBA1C levels were not incorporated, initial renal function status was missing, and for the diagnosis of AKI, urine output criteria were not used. Finally, the requirement for dialysis was not assessed using defined criteria. This absence of differences between these 2 groups may be explained by these confounding factors.
Very few studies have examined concurrent AKI, CKD, and recurrent AKI in this group of patients. [ 18 ] Table Table1 1 summarizes studies related to this topic.
Few studies assessed AKI incidences and outcomes in diabetic patients.
AKI = acute kidney injury, DM = diabetes mellitus.
3. Risk factors for AKI
3.1. diabetes and non-modifiable factors.
Girman et al [ 20 ] reported that diabetes alone was still associated with a higher risk of acute kidney failure, even after accounting for other risk factors, such as chronic kidney disease. Additional risk factors for AKI were increasing age, chronic kidney disease, systemic hypertension, previous history of AKI, and congestive cardiac failure. The combination of type 2 diabetes with congestive cardiac failure or systemic hypertension further increases the risk of AKI. In a previous study, elderly patients with heart failure had a 3.37-fold higher risk of AKI than those without heart failure, whereas hypertension was linked to a 1.94-fold higher risk of acute kidney injury. [ 30 ] Acute kidney injury occurred in 21% of patients in a cohort study admitted with congestive heart failure as their primary diagnosis. [ 31 ] preexisting proteinuria, hypertension, and diabetes mellitus were all independent AKI risk variables revealed in the study by Hsu et al [ 11 ]
3.2. Proteinuria and lower eGFR
According to Prabhu et al [ 22 ] , there was a correlation between AKI incidence and lower baseline eGFR, and higher proteinuria. With every 1 g rise in proteinuria, they have revealed that AKI risk was increased by 15.8%. Moseu et al came to the same conclusion regarding the correlation of AKI incidence with lower eGFR and albuminuria. [ 19 ] A large cohort study showed moderate to high proteinuria was a risk factor for AKI among all eGFR groups in hospitalized patients with AKI. [ 32 ]
3.3. Hypoglycemic agents
In comparison, nondiabetic patients, surgical patients with diabetes, and those taking oral antidiabetic medications had a 30% higher chance of developing acute renal failure following surgery, while those taking insulin had a 70% higher risk. [ 33 ]
Drug-induced AKI represents 20% of all etiologies. [ 34 ] Patho physiological mechanism depends on the type of drug involved. [ 34 ] ACE/ARB (angiotensin-converting enzyme/angiotensin receptor blocker) were the main causes of AKI contributing to 35% of cases due to their increased use in diabetic patients. This risk was even higher in patients with congestive heart failure, volume depletion, diuretics, nonsteroidal anti-inflammatory drugs (NSAIDs), and bilateral renal artery stenosis. Aminoglycoside (gentamicin) and NSAIDs contributed to 16% followed by statins (10%), antitubercular agents (rifampicin), and ifosfamide 6% and 3% respectively. Glomerular filtration rate and renal blood flow are decreased as a result of the suppression of prostaglandin production due to NSAIDs. They also reported dehydration and intravenous rehydration as prognostic factors in their study. [ 35 ]
The histological lesion of AKI caused by diuretics may primarily manifest as tubular epithelial cell vacuolation. Risk is even higher in a combination of other drugs that is, NSAIDs, antibiotics, ACEi, and contrast. [ 36 ] Diabetic patients who used hydrochlorothiazide (HCT) frequently experienced renal events (decline in eGFR > 30%), which affected about 20% of individuals as shown in a retrospective study. [ 37 ] Similarly, another study showed that diuretic-associated AKI patients had a higher rate of comorbidities (DM, CVD, CKD, hypertension) as compared to the non-diuretic AKI group. In the diuretic-induced AKI group, 27.5% was caused by diuretics only and 29.8% was caused by the combination of diuretics with other drugs. [ 36 ]
AKI hospitalizations in the US have recently increased considerably, from 35,000 in 1979 to 650,000 in 2002. This increase was attributed to the increasing drug consumption by the elderly, and various comorbidities. [ 38 ] Similarly, from 1992 to 2001, AKI incidence among Medicare beneficiaries increased by 11% annually, with higher rates seen in the elderly, men, and African-Americans. [ 39 ]
Although it is believed that the use of ACE/ARB is linked to acute renal failure, it can be challenging to interpret published studies because those who are most at risk for AKI may also be the ones who are most likely to receive treatment with angiotensin-converting enzyme/ARBs. [ 40 ]
3.5. Dehydration
Extracellular volume depletion due to glycosuria because of uncontrolled diabetes especially in pediatric patients leads to prerenal AKI. [ 41 ] The combined effect of uncontrolled diabetes along with prerenal AKI may cause intrinsic renal AKI, characterized by renal parenchymal damage and tubular necrosis. [ 42 ]
3.6. Sepsis
Along with dysfunctional immune systems both humoral and cell-mediated, increase neutrophil dysfunction also contributes to an increased risk of sepsis. [ 22 ] Sepsis was the primary cause of AKI in a retrospective study done by Prabhu et al [ 22 ] In addition, they showed that there was a higher eGFR decline secondary to sepsis-related AKI as compared to other etiologies. Diabetes mellitus has been demonstrated to be an independent risk factor in a recent meta-analysis of sepsis-related AKI. [ 43 ]
3.7. Contrast
Diabetic patients, particularly those who developed DN, are more prone to contrast-induced injury. Diabetes and contrast-induced acute kidney injury (CI-AKI) are mutually causative, causing kidney function to deteriorate further. Renal hypoxia, generation of reactive oxygen species, and increased oxidative stress in diabetic patients lead to vascular constriction due to vasoactive substances. Immunological changes in diabetic patients also contribute to contrast-induced AKI. Signaling pathways that is, inflammation, reactive oxygen species production, and apoptosis related to both diabetes and contrast-induced AKI. [ 44 ] Due to impaired nitro vasodilation, increased endothelin synthesis, and hyperresponsiveness to adenosine-related vasoconstriction, peritubular blood flow may also be affected. [ 45 ] According to data, the incidence of Contrast-induced AKI ranges from 5.7% to 29.4% in diabetes patients and is approximately 13% in nondiabetic patients. [ 46 ] A recent meta-analysis showed that diabetes is associated with a higher risk of CI-AKI. Moreover, the subgroup of DM patients with CKD had a greater predictive effect of elevated CI-AKI but this correlation was not significant in the subgroup of patients without CKD. [ 47 ]
Table Table2 2 summarizes the causes of AKI in diabetic patients.
Risk factors of AKI in diabetic patients.
AKI = acute kidney injury, NSAIDs = nonsteroidal anti-inflammatory drugs.
4. Pathophysiologic mechanisms of AKI in patients with diabetes
The pathophysiologic mechanisms causing diabetes-related kidney damage are multifactorial. (Fig. (Fig.1) 1 ) It has been hypothesized that structural and functional alterations in the renal vasculature and the tubular epithelial cells increase the cytokines and chemokines generation, which produce inflammation, ischemia, and isolated proximal tubulopathy. [ 48 , 49 ]

Pathophysiological mechanism of diabetes-induced kidney damage.
Endothelial cell dysfunction is one of the main mechanisms underlying DN. Diabetic kidneys are known to produce less nitric oxide (NO), which is produced by the enzyme endothelial nitric oxide synthase (eNOS). Because of diabetes’s distorted NO metabolism, the renal vasculature is more vulnerable to stimuli that cause vasoconstriction. [ 3 ]
It is believed that in uncontrolled diabetes, renal vasculature dysregulation is the primary factor contributing to glomerular hyperfiltration. [ 50 , 51 ] Persistent glomerular hyperfiltration causes intraglomerular hypertension, followed by glomerulosclerosis, which causes a progressive decline in kidney function and eventually DKD. [ 50 , 51 ]
In the case of prerenal AKI, when the body depends on variations in renal vascular resistance to maintain blood pressure, a dysregulation in normal renal vascular tone may hasten the kidney damage. [ 52 , 53 ] The absence of a suitable vascular counterregulatory response to sustain kidney blood flow can also significantly worsen kidney hypoperfusion. [ 50 , 51 ]
Chronic and acute renal damage related to diabetes may be exacerbated by hyperuricemia. [ 54 ] It has been demonstrated that hyperuricemia can cause crystal-mediated and crystal-independent nephropathy, glomerular injury, and tubulointerstitial involvement. [ 55 ] It is crucial to remember that hyperuricemia could signify dehydration, which can directly cause renal injury.
Persistent hyperglycemia, which is related to prolonged ICU stays and an increased risk of AKI is another pathophysiologic pathway that results in CKD and eventually ESRD. [ 3 ] Apoptosis of endothelial cells, vascular rarefaction and hypoxia, mitochondrial dysfunction, proximal tubular disorder, podocyte disorder, podocyte apoptosis, and autophagy due to diabetes have all been shown in laboratory studies. [ 3 ]
5. AKI in diabetic versus nondiabetic patients
Diabetic patients are at higher risk of AKI than nondiabetic patients, which can be attributed to diabetes, chronic kidney disease, hyperglycemic crisis, drugs that is, ACE inhibitors and sodium-glucose cotransporter-2 (SGLT2) inhibitors, associated cardiovascular disease and heart failure, and previous AKI episodes. [ 56 ] Girman et al [ 20 ] in a retrospective cohort showed that diabetic patients were 8 times more likely to have incident acute renal failure than nondiabetic patients. There have been 2 retrospective analyses, both of which had conflicting findings. [ 10 , 57 ] Diabetic patients had less severe AKI, recovery to baseline renal function and the proportion of patients developing progressive CKD was lower in the diabetic group as shown by Johns et al [ 10 ] However, the 10-year retrospective analysis done by Xin S et al showed that the recurrence rate of AKI was higher in the diabetic group than in the nondiabetic group. [ 57 ] Between diabetes and nondiabetic groups, mortality was comparable in both retrospective analyses.
6. Diabetes and cardiorenal syndrome
Cardiorenal syndrome (CRS) is a disease affecting the heart and kidneys simultaneously. T2DM is a significant risk factor for the development of CRS; the National Health and Nutrition Examination Survey in the USA found a strong association between type 2 CRS and T2DM. [ 58 ] Moreover, being a systemic disorder affecting the heart and kidneys, and it is also associated with type 4 and type 5 CRS. [ 59 ] SGLT 2 inhibitors were implicated in CRS due to both renal and cardioprotective effects. Reno protective effects of SGLT2 inhibitors in AKI contributed by increased vascular endothelial growth factor A expression, increase vasodilatation due to NO, and decrease renal fibrosis. [ 60 ] Regardless of the presence of atherosclerotic CVD or a history of heart failure, these drugs decrease the hospitalization rate for heart failure and the progression of renal illness. [ 61 ] EMPA-REG OUTCOME (Empagliflozin cardiovascular outcome event trial in type 2 diabetes mellitus), DECLARE-TIMI 58, CANVAS (Canagliflozin Cardiovascular Assessment Study), and CREDENCE (Canagliflozin and Renal Events in Diabetes with Established Nephropathy) are the 4 major trials in diabetic patients showed positive cardiovascular and renal outcomes. [ 62 – 65 ] In December 2016 there was an FDA alert regarding the use of canagliflozin and dapagliflozin. [ 66 ] However, this increased risk of AKI with SGLT2 inhibitors was not supported by studies. [ 67 ] Meta-analysis showed the protective effect of SGLT2 inhibitors with AKI, primarily driven by empagliflozin. [ 68 ]
These drugs should not be started in CRS 1 and 3 but may be continued with close hemodynamic and renal function monitoring. SGLT2 inhibitors are preferred drugs in CRS types 2, 4, and 5 for glycaemic, as well as metabolic, control. [ 69 ]
7. Preventive strategies and implications
In AKI, there is no universal therapy for AKI. The primary goals of treatment are to address underlying causes, such as dehydration, avoiding nephrotoxic drugs, fluid, and electrolyte management, and renal replacement therapy. [ 70 ]
Obese patients have glomerulomegaly, increase renal blood flow, hyperfiltration, and higher albuminuria despite the absence of hypertension. [ 71 ] Moreover, sleep apnea in obese patients causes hypoxic episodes contributing to renal impairment. [ 72 ] Hence weight control is an important preventive aspect in terms of decreasing renal injury.
Renin-angiotensin-aldosterone system inhibitors have been shown to attenuate proteinuria and continue to be the cornerstone of current therapeutic methods. [ 73 , 74 ] As patients with T2DM has more significantly greater urinary albumin-to-creatinine ratios than patients with T1DM even after adjustment for all known risk factors for diabetic kidney disease, as shown in the SEARCH study, hence RASS inhibitors are indicated in diabetic patients treatment frequently. [ 75 ] However, these drugs have nephrotoxic effects directly or indirectly by affecting renal hemodynamic function. [ 72 , 74 ]
Many antihyperglycemic agents, including metformin, thiazolidinediones, dipeptidyl peptidase inhibitors, Glucagon-like peptide agonists, and SGLT2 (Sodium-glucose Cotransporter-2) inhibitors, also have nephroprotective properties in addition to glucose-lowering effects. [ 76 – 78 ] However, accumulation of metformin in case of impaired eGFR (e.g., 30–60 mL/minutes/1.73 m2) causes type B lactic acidosis and toxicity by impairing mitochondrial function. Thiazolidinediones, such as pioglitazone, have been shown to decrease proteinuria in a large meta-analysis. [ 46 ] However, no randomized controlled trials showed renal protective effects of thiazolidinediones. [ 79 ] Role of dipeptidyl peptidase inhibitors and glucagon-like peptide 1 receptor agonist as nephroprotective agents are controversial. These have been shown to have nephroprotective effects in some studies. [ 76 , 80 ] However their effects on eGFR were uncertain as shown in a recent Cochrane review. [ 80 ] SGLT2 inhibitors were found to have a nephroprotective effect as shown in DECLARE-TIMI 58 and EMPA-REG OUTCOME trials, but in the Cochrane review, it did not show any effect on AKI risk. [ 80 – 82 ]
Some drugs have been evaluated in animal models and may represent future therapeutic options for AKI prevention, such as mineralocorticoid receptor antagonists, endothelin receptor antagonists, peroxisome proliferator-activated receptors agonists, and phosphodiesterase inhibitors. In animal models, finerenone also decreases the progression of AKI to CKD, and hence it can be an excellent therapeutic option in AKI to prevent long-term complications. [ 83 ]
8. Conclusion
In summary, AKI is a complication of diabetes mellitus. It increases the risk of further episodes of AKI, progression to chronic kidney disease, end-stage renal disease, cardiac and cerebrovascular events, and all-cause morbidity and mortality. Additionally, diabetes is the risk factor for AKI irrespective of underlying CKD. Current strategies should focus on its identification and mitigation, reducing proteinuria, weight control, fluid management, removal of precipitant factors (drugs, sepsis, contrast), and other supportive measures to improve AKI outcomes. Many glucose-lowering drugs (SGLT2 inhibitors) have nephroprotective against AKI in patients with diabetes in addition to their antidiabetic effects. A promising new approach to treating AKI and CKD using novel classes of medications that target renal hemodynamic dysfunction in diabetic patients. In the interim, healthcare professionals need to be aware of the risks and effects of AKI in patients with diabetes.
Author contributions
Conceptualization: Amninder Kaur, Gaurav Shekhar Sharma, Damodar Kumbala.
Supervision: Gaurav Shekhar Sharma.
Validation: Gaurav Shekhar Sharma, Damodar Kumbala.
Visualization: Amninder Kaur, Damodar Kumbala.
Writing – original draft: Amninder Kaur.
Writing – review & editing: Amninder Kaur, Gaurav Shekhar Sharma.s
Abbreviations:
The authors have no funding and conflicts of interest to disclose.
Data sharing not applicable to this article as no datasets were generated or analyzed during the current study.
How to cite this article: Kaur A, Sharma GS, Kumbala DR. Acute kidney injury in diabetic patients: A narrative review. Medicine 2023;102:21(e33888).
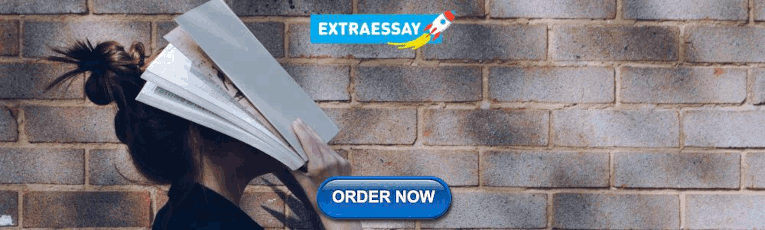
IMAGES
VIDEO
COMMENTS
A 68-year-old man was admitted to the hospital with fever, shortness of breath, and acute kidney injury. Testing of a nasopharyngeal swab for SARS-CoV-2 RNA was positive. Respiratory failure and hy...
Adult II Case Study-Acute Kidney Injury. Patient Profile A 78 year-old woman with a long history of hypertension, heart failure, and coronary artery surgery 12 years ago. She was admitted 2 days ago for heart failure exacerbation. Despite being treated with diuretics, her urinary output is decreased.
A 15-year-old girl presented with acute kidney injury. Eight days earlier, bloody diarrhea and abdominal cramping had developed. On admission, the creatinine level was 7.71 mg per deciliter, and an...
PowerPoint Presentation. Case study. Patient David Smith. Situation. 68 year old gentleman admitted to Emergency Department with collapse. Background. Patient states feeling unwell for last 5 days and hasn't been eating and drinking as much as usual. Past medical history.
Acute kidney injury (AKI) is an abrupt and usually reversible decline in the glomerular filtration rate (GFR). Patients with AKI must be evaluated promptly to determine cause. Different disorders can BE associated with AKI, and biopsy is the most accurate instrument for diagnosis of different types of diseases. We report a case of 69-year-old ...
Abstract. Exercise-induced acute kidney injury (EIAKI) frequently develops in patients with renal hypouricemia (RHUC). However, several cases of RHUC with acute kidney injury (AKI) but without intense exercise have been reported. We encountered a 15-year-old male with RHUC who experienced AKI. He reported no episodes of intense exercise and displayed no other representative risk factors of ...
Acute kidney injury (AKI), previously called acute renal failure (ARF), denotes a sudden and often reversible reduction in kidney function, as measured by glomerular filtration rate (GFR).[1][2][3] However, immediately after a renal insult, blood urea nitrogen (BUN) or creatinine (Cr) levels may be within the normal range, and the only sign of AKI may be a decline in urine output. AKI can lead ...
1. Introduction. Acute kidney injury (AKI) is a frequent diagnosis with an incidence that varies from 5.0% to 7.5% in hospitalized patients and that reaches up to 50-60% in critically ill patients [1,2,3,4,5,6].AKI is characterized by an acute decrease in renal function that can be multifactorial in its origin and is associated with complex pathophysiological mechanisms [1,7].
Acute kidney injury (AKI) is defined by a sudden loss of excretory kidney function. AKI is part of a range of conditions summarized as acute kidney diseases and disorders (AKD), in which slow ...
Comprehensive case study: acute kidney injury. Comprehensive case study: acute kidney injury. Comprehensive case study: acute kidney injury Medsurg Nurs. 2013 Nov-Dec;22(6):400-2. Author Stephanie Gedeon 1 Affiliation 1 Henrico Doctors' Hospital, Richmond, VA, USA. PMID: 24600939 No abstract available ...
The incidence of acute kidney injury has increased in recent years, both in the community and in hospital settings. 1, 2 The estimated incidence of acute kidney injury is two to three cases per ...
We're going to go through a case study for acute kidney injury together. Let's get started. In this scenario, our patient is Ms. Barkley. She's thin and frail, 64 years old and presents from a nursing home for acute abdominal pain as well as nausea and vomiting that she's been experiencing for the last two days.
Case Study Objectives. Analyze and interpret clinical data and patient assessments to identify signs and symptoms of acute kidney injury (AKI) in a real-life patient scenario. Apply critical thinking skills to recognize the physiological mechanisms contributing to the development of AKI, considering factors such as dehydration, contrast dye ...
Background : Acute kidney injury (AKI) is associated with substantial mortality. In this case-control study, we analyzed the impacts of AKI and chronic kidney disease (CKD) on outcomes in a group of 323 patients with severe COVID-19. The correlation of clinical and laboratory data with AKI and …
Acute kidney injury (AKI) is associated with poor outcome both in critically ill patients and after major surgery.1 The occurrence of AKI has been associated with poor short-term and long-term outcome, increased risk of chronic renal failure, and increased risk of death.2 Several risk factors of postoperative AKI have been identified, and may help identifying patients with the highest risk of ...
Hollinger, A. et al. Proenkephalin A 119-159 (Penkid) is an early biomarker of septic acute kidney injury: the kidney in sepsis and septic shock (Kid-SSS) study. Kidney Int. Rep. 3 , 1424-1433 ...
Acute Kidney Injury. Case Study # Patient Profile. A. is a 70-year-old white woman who presented to the emergency department because of a 4- day history of increased shortness of breath and generalized weakness. A. stated that she has been able to do her daily chores at home independently, but for the past few days, it was getting difficult for ...
Acute kidney injury case study with questions and answers Single best answer question based on renal clinical case: Single best answer question 1. A 50 year old alcoholic male presents with sepsis secondary to klebsiella pneumonia. His background includes IHD, previous pneumonia, hypercholesterolaemia and hypertension. Medications include: furosemide, enalapril, aspirin, clopidogrel, co ...
Official Ninja Nerd Website: https://www.ninjanerd.orgNinja Nerds!During this lecture Professor Zach Murphy will be discussing our 14th case study with you a...
Background. Currently, there is an unprecedented surge in the demand for intensive care resources throughout the world due to the COVID-19 pandemic. 1 2 Little is known about rhabdomyolysis and acute kidney injury (AKI) in the context of the COVID-19 infection in the intensive care setting. 3 4 Recent reports suggest that AKI during COVID-19 ...
Acute kidney injury (AKI), also known as Acute Renal Failure (ARF) occurs when the kidneys lose their filtering ability resulting in the build-up of waste products in the blood.This condition develops rapidly, in hours or days, and is common in critically ill patients.. This condition affects other organs in the body if not treated promptly and can be life-threatening.
Acute kidney injury (AKI) in intensive care units (ICUs) is an independent risk factor for death. Reported mortality rates from AKI with renal replacement therapy (RRT; 40-55%) are higher than the mortality rates due to ICU-related myocardial infarction (20%), sepsis without AKI (15-25%), or acute respiratory distress syndrome requiring mechanical ventilation (30-40%) [].
Acute kidney injury (AKI) is defined by sudden-onset renal cell injury and functional impairment. AKI is diagnosed in over 13 million people globally each year, but subclinical and, consequently, additional undiagnosed cases are common, especially in otherwise healthy adults (Petejova and Martinek, 2014; Zou et al., 2022).
The nurse should monitor Mr. Brown for possible fluid retention related to acute kidney injury. Can you identify indicators of fluid volume excess? - Weight Gain. - Distended Neck Veins. Study with Quizlet and memorize flashcards containing terms like Prerenal Acute Kidney Injury, Intrarenal Acute Kidney Injury, Postrenal Acute Kidney Injury ...
Sepsis and acute kidney injury (AKI) are common severe diseases in the intensive care unit (ICU). This study aimed to estimate the attributable mortality of AKI among critically ill patients with sepsis and to assess whether AKI was an independent risk factor for 30-day mortality. The information we used was derived from a multicenter prospective cohort study conducted in 18 Chinese ICUs ...
Acute kidney injury (AKI) is a frequently encountered complication in hospitalized patients with acute illness, ... Taiwan approved this case-control study on February 7, 2023 (approved number, N201703004). Before any analyses, all patient data were anonymized.
The drugs that mostly frequently induce acute kidney injury: a case − noncase study of a pharmacovigilance database. ... The drugs that mostly frequently induce acute kidney injury: a case − noncase study of a pharmacovigilance database. Br J Clin Pharmacol, 83: 1341-1349. doi: 10.1111/bcp.13216. ...
The French clinical case reported in the correspondence concerns a 26-year-old woman with no prior health history who had three episodes of acute renal damage 1 year apart.
Acute kidney injury (AKI), defined as a rapid increase in serum creatinine, decrease in urine output, or both, is a common clinical syndrome caused by multiple factors, including renal ischemia, sepsis, toxic effects from drugs, and pigment-related injury from myoglobin or hemoglobin [1, 2].According to a recent statistical report, AKI occurs in approximately 13.3 million people per year ...
In a previous study, elderly patients with heart failure had a 3.37-fold higher risk of AKI than those without heart failure, whereas hypertension was linked to a 1.94-fold higher risk of acute kidney injury. Acute kidney injury occurred in 21% of patients in a cohort study admitted with congestive heart failure as their primary diagnosis.