- Share full article
Advertisement
Supported by
Personal Health
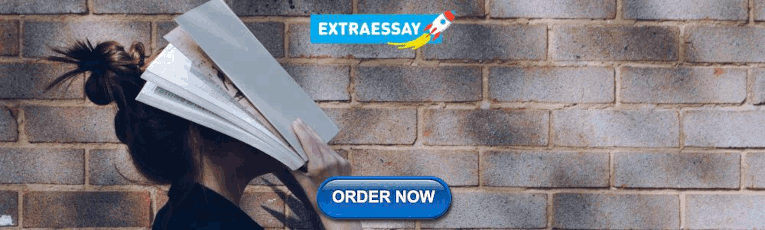
Why Nearsightedness Is on the Rise in Children
Decreased exposure to outdoor light appears to be a major factor in rising rates of myopia in young people around the world.

By Jane E. Brody
Look and you shall see: A generation of the real-life nearsighted Mr. Magoos is growing up before your eyes. A largely unrecognized epidemic of nearsightedness, or myopia, is afflicting the eyes of children.
People with myopia can see close-up objects clearly, like the words on a page. But their distance vision is blurry, and correction with glasses or contact lenses is likely to be needed for activities like seeing the blackboard clearly, cycling, driving or recognizing faces down the block.
The growing incidence of myopia is related to changes in children’s behavior, especially how little time they spend outdoors, often staring at screens indoors instead of enjoying activities illuminated by daylight. Gone are the days when most children played outside between the end of the school day and suppertime. And the devastating pandemic of the past year may be making matters worse.
Susceptibility to myopia is determined by genetics and environment. Children with one or both nearsighted parents are more likely to become myopic. However, while genes take many centuries to change, the prevalence of myopia in the United States increased from 25 percent in the early 1970s to nearly 42 percent just three decades later. And the rise in myopia is not limited to highly developed countries. The World Health Organization estimates that half the world’s population may be myopic by 2050.
Given that genes don’t change that quickly, environmental factors, especially children’s decreased exposure to outdoor light, are the likely cause of this rise in myopia, experts believe. Consider, for example, factors that keep modern children indoors: an emphasis on academic studies and their accompanying homework, the irresistible attraction of electronic devices and safety concerns that demand adult supervision during outdoor play. All of these things drastically limit the time youngsters now spend outside in daylight, to the likely detriment of the clarity of their distance vision.
Recent research suggests that months of Covid-induced confinement may be hastening myopia’s silent progression among young children. A Canadian study that examined children’s physical activity, outdoor time, screen time and social media use during the Covid lockdown in early 2020 found that 8-year-olds spent an average of more than five hours a day on screens for leisure, in addition to screen time needed for schoolwork.
This report and a new study of school-aged children in China after five months of Covid-19 home confinement informed the title of an editorial, “ 2020 as the Year of Quarantine Myopia, ” in the Jan. 14 issue of JAMA Ophthalmology. Researchers from Emory University in Atlanta, the University of Michigan in Ann Arbor and Tianjin Medical University Eye Hospital in Tianjin, China, described a substantial decline in the visual acuity among 123,535 elementary school children following school closures last year from January until June.
Compared to the results of previous annual screenings, the ability to see distant objects clearly had fallen precipitously, especially among those ages 6 to 8. The children became far more myopic than expected, based on changes in acuity that were measured at the start of the school years 2015 through 2019. But a similarly dramatic drop in acuity among older children was not found.
“Given the fact that the younger children were assigned fewer online learning tasks than the older ones, it is unlikely that rapidly progressing myopia in younger children was caused by more intense screen time or near work,” like reading, typing, doing homework or playing video games , the research team wrote in JAMA Ophthalmology. Rather, a lack of exposure to outdoor light is the more likely explanation.
As the editorial writers from Erasmus University Medical Center in the Netherlands suggested, “young children may be more sensitive to myopic triggers from the environment.” An earlier eye study among children in Sydney, Australia, also found that only the younger ones who became myopic had spent more time on near work rather than being out in daylight.
Although many people have long believed that excessive reading fosters nearsightedness in children, current thinking is that too much time spent indoors has the greater effect and likely accounts for any apparent association between close work or screen time and myopia.
Dr. Neil M. Bressler, an ophthalmologist affiliated with Johns Hopkins Medical Institutions, said that the high intensity of outdoor light has an important influence on the shape of the eye, which in turn affects whether images are seen clearly.
To be in focus, light rays from an image have to converge on the retina. In myopic eyes, the convergence occurs in front of the retina, and a corrective lens is needed to redirect incoming rays so that distant objects are in focus.
Most children are born slightly farsighted. Their eyes are shaped like partly deflated balls, causing images to converge behind the retina. But as they get older, their eyes elongate to form a sphere, permitting images to converge directly on the retina. However, if elongation fails to stop at some point, the eyes become more oval and images then converge in front of the retina, the definition of myopia. Outdoor light stimulates the release of dopamine that may slow elongation of the eye, Dr. Bressler said.
Although the rise of myopia is happening worldwide, the epidemic is raging in east and Southeast Asia, where 80 percent to 90 percent of high school children are now myopic.
Concern over the increasing prevalence of myopia goes beyond a growing need for glasses, contact lenses or, for those so inclined and who can afford it, laser treatment to redirect images by changing the shape of the cornea. In general, people with myopia are more likely to develop sight-threatening complications later in life like cataracts, glaucoma and degeneration of the macula, the center of the retina.
If the condition becomes extreme, Dr. Bressler said, “it can be tough to correct.” The eye becomes stretched, the retina can spread and form scar tissue and the gel in the center of the eye can become stuck to the sides of the eye, causing retinal tears or detachment, he explained.
Such risks are stimulating studies of treatments that might prevent myopia from becoming pathological. One method under study is the use of multifocal contact lenses with high magnifying power to try to slow progression of myopia in children. Another approach, currently considered more promising, is the use of atropine eye drops to minimize undue elongation of the eye. A third approach, called orthokeratology, involves wearing contact lenses at night to change the shape of the cornea, make the edges of the eye more farsighted and perhaps slow the eye’s elongation.
“The pandemic has put fuel on the fire,” Dr. Bressler said, “but we don’t have a treatment yet.” Currently, the most effective preventive may be for young children to spend less time on screens and a lot more time outdoors.
Jane Brody is the Personal Health columnist, a position she has held since 1976. She has written more than a dozen books including the best sellers “Jane Brody’s Nutrition Book” and “Jane Brody’s Good Food Book.” More about Jane E. Brody
The Mysteries and Wonders of Our DNA
Women are much more likely than men to have an array of so-called autoimmune diseases, like lupus and multiple sclerosis. A new study offers an explanation rooted in the X chromosome .
DNA fragments from thousands of years ago are providing insights into multiple sclerosis, diabetes, schizophrenia and other illnesses. Is this the future of medicine ?
A study of DNA from half a million volunteers found hundreds of mutations that could boost a young person’s fertility and that were linked to bodily damage later in life.
In the first effort of its kind, researchers now have linked DNA from 27 African Americans buried in the cemetery to nearly 42,000 living relatives .
Environmental DNA research has aided conservation, but scientists say its ability to glean information about humans poses dangers .
That person who looks just like you is not your twin. But if scientists compared your genomes, they might find a lot in common .
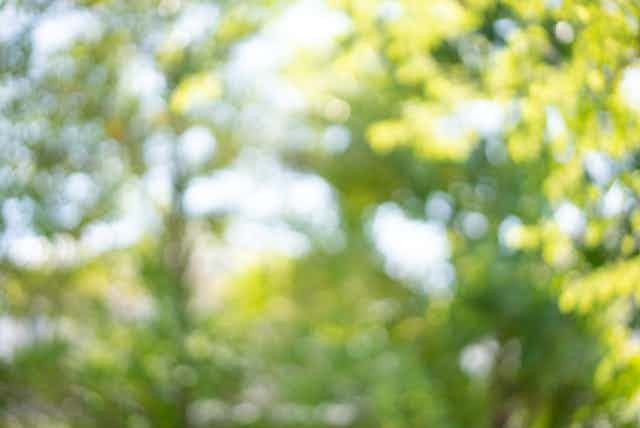
Nearsightedness is at epidemic levels – and the problem begins in childhood
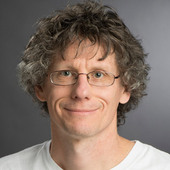
Professor of Psychology, Visual Perception, Rochester Institute of Technology
Disclosure statement
Andrew Herbert receives funding from NSF.
Rochester Institute of Technology provides funding as a member of The Conversation US.
View all partners
Myopia, or the need for corrected vision to focus or see objects at a distance, has become a lot more common in recent decades. Some even consider myopia , also known as nearsightedness, an epidemic.
Optometry researchers estimate that about half of the global population will need corrective lenses to offset myopia by 2050 if current rates continue – up from 23% in 2000 and less than 10% in some countries .
The associated health care costs are huge. In the United States alone, spending on corrective lenses, eye tests and related expenses may be as high as US$7.2 billion a year .
What explains the rapid growth in myopia?
I’m a vision scientist who has studied visual perception and perceptual defects. To answer that question, first let’s examine what causes myopia – and what reduces it.
How myopia develops
While having two myopic parents does mean you’re more likely to be nearsighted, there’s no single myopia gene . That means the causes of myopia are more behavioral than genetic.
Optometrists have learned a great deal about the progression of myopia by studying visual development in infant chickens . They do so by putting little helmets on baby chickens. Lenses on the face of the helmet cover the chicks’ eyes and are adjusted to affect how much they see.
Just like in humans, if visual input is distorted, a chick’s eyes grow too large, resulting in myopia . And it’s progressive. Blur leads to eye growth, which causes more blur, which makes the eye grow even larger, and so on.
Two recent studies featuring extensive surveys of children and their parents provide strong support for the idea that an important driver of the uptick in myopia is that people are spending more time focusing on objects immediately in front of our eyes, whether a screen, a book or a drawing pad. The more time we spend focusing on something within arm’s length of our faces, dubbed “near work,” the greater the odds of having myopia.
So as much as people might blame new technologies like smartphones and too much “screen time” for hurting our eyes, the truth is even activities as valuable as reading a good book can affect your eyesight.
Outside light keeps myopia at bay
Other research has shown that this unnatural eye growth can be interrupted by sunlight.
A 2022 study, for example, found that myopia rates were more than four times greater for children who didn’t spend much time outdoors – say, once or twice a week – compared with those who were outside daily. At the same time, kids who spent more than three hours a day while not at school reading or looking at a screen close-up were four times more likely to have myopia than those who spent an hour or less doing so.
In another paper, from 2012, researchers conducted a meta-analysis of seven studies that compared duration of time spent outdoors with myopia incidence. They also found that more time spent outdoors was associated with lower myopia incidence and progression. The odds of developing myopia dropped by 2% for each hour spent outside per week.
Other researchers have reported similar effects and argued for much more time outdoors and changes in early-age schooling to reduce myopia prevalence.
What’s driving the epidemic
That still doesn’t explain why it’s on the rise so rapidly.
Globally, a big part of this is due to the rapid development and industrialization of countries in East Asia over the last 50 years. Around that time, young people began spending more time in classrooms reading and focusing on other objects very close to their eyes and less time outdoors.
This is also what researchers observed in the North American Arctic after World War II, when schooling was mandated for Indigenous people. Myopia rates for Inuit went from the single digits before the 1950s to upwards of 70% by the 1970s as all children began attending schools for the first time.
Countries in Western Europe, North America and Australia have shown increased rates of myopia in recent years but nothing approaching what has been observed recently in China, Japan, Singapore and a few other East Asian countries . The two main factors identified as leading to increased myopia are increased reading and other activities that require focusing on an object close to one’s eyes and a reduction in time spent outdoors .
The surge in myopia cases will likely have its worst effects 40 or 50 years from now because it takes time for the young people being diagnosed with nearsightedness now to experience the most severe vision problems.
Treating myopia
Fortunately, just a few minutes a day with glasses or contact lenses that correct for blur stops the progression of myopia , which is why early vision testing and vision correction are important to limit the development of myopia. Eye checks for children are mandatory in some countries, such as the U.K. and now China , as well as most U.S. states .
People with with high myopia, however, have increased risk of blindness and other severe eye problems , such as retinal detachment, in which the retina pulls away from the the back of the eye. The chances of myopia-related macular degeneration increase by 40% for each diopter of myopia . A diopter is a unit of measurement used in eye prescriptions.
But there appear to be two sure-fire ways to offset or delay these effects: Spend less time focusing on objects close to your face, like books and smartphones, and spend more time outside in the bright, natural light. Given the first one is difficult advice to take in our modern age, the next best thing is taking frequent breaks – or perhaps spend more time reading and scrolling outside in the sun.
- Vision problems

Project Offier - Diversity & Inclusion

Senior Lecturer - Earth System Science

Sydney Horizon Educators (Identified)

Deputy Social Media Producer

Associate Professor, Occupational Therapy
Thank you for visiting nature.com. You are using a browser version with limited support for CSS. To obtain the best experience, we recommend you use a more up to date browser (or turn off compatibility mode in Internet Explorer). In the meantime, to ensure continued support, we are displaying the site without styles and JavaScript.
- View all journals
- My Account Login
- Explore content
- About the journal
- Publish with us
- Sign up for alerts
- Open access
- Published: 15 December 2022
Myopia: why the retina stops inhibiting eye growth
- Barbara Swiatczak 1 &
- Frank Schaeffel 1 , 2 , 3
Scientific Reports volume 12 , Article number: 21704 ( 2022 ) Cite this article
7560 Accesses
6 Citations
51 Altmetric
Metrics details
- Neuroscience
- Translational research
In myopia, the eye grows too long, and the image projected on the retina is poorly focused when subjects look at a distance. While the retina normally controls eye growth by visual processing, it seems to give up during myopia development. But what has changed? To determine whether the sharp image is in front or behind the retinal plane, a comparison of image sharpness in red and blue would provide a reliable cue because focal planes are about 1.3 D apart due to longitudinal chromatic aberration (LCA). However, up to now, it could not be demonstrated that the retina does, in fact, such a comparison. We used a new approach: movies were digitally filtered in real time to present either the blue channel of the RGB color format unfiltered while green and red were blurred (“blue in focus”), or the red channel was unfiltered while green and blue were blurred (“red in focus”) accordingly to the human LCA function. Here we show that, even though filtered movies looked similar, eyes became significantly shorter when the movie was sharp in the red plane but became longer when it was presented sharp in the blue plane. Strikingly, the eyes of young subjects who were already myopic did not respond at all—showing that their retina could no longer decode the sign of defocus based on LCA. Our findings resolve a long-standing question as to how the human retina detects the sign of defocus. It also suggests a new non-invasive strategy to inhibit early myopia development: keeping the red image plane on a computer screen sharp but low pass filtering the blue.
Similar content being viewed by others
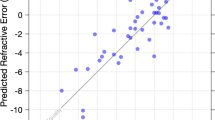
Altered spatial summation optimizes visual function in axial myopia
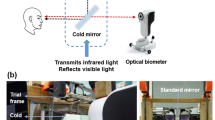
The time course of the onset and recovery of axial length changes in response to imposed defocus
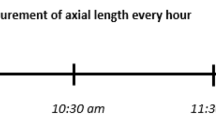
Effect of short-term peripheral myopic defocus on ocular biometrics using Fresnel “press-on” lenses in humans
Introduction.
In the past decades, myopia became the most frequent developmental disorder in the eyes of young people in many countries of the world, now affecting more than two billion individuals 1 . In addition to poor visual acuity at a distance, the exaggerated expansion of the eyeball leads to progressive thinning of the tissue layers in the back of the eye which increases the risk of retinal degeneration, retinal detachment, and other ocular pathologies that may lead to blindness already in the mid of the life span 2 . Myopia typically starts to develop at school age but tends to progress during the following years. Why the eye deviates from its normally perfectly controlled growth path is intensively studied, but largely this question is still unresolved. Extended periods of near work and reading were traditionally associated with myopia development but a causal link or an explanation of a potential mechanism is lacking until today (reviews: 3 , 4 ). A major step in the understanding of myopia was that axial eye growth is controlled almost exclusively by the retina 5 , 6 . Even more striking, it was found in the chicken that the retina is able to detect the sign of defocus also when only one viewing distance is available and the retinal image is heavily blurred 6 , 7 , 8 .
Less time spent outdoors is one of the main risk factors of myopia development. It has been shown in prospective study that children who spent outdoors 5 h more per week remained non-myopic when compared with children who became myopic 9 . The obvious difference between indoor and outdoor environments is light intensity. And indeed, it has been shown that exposure to bright light as found outdoors can delay the onset of myopia and perhaps also reduce its progression due to increasing dopamine level in the retina (i.e. 10 ). Recently, interest was shifted towards the effects of spectral composition of light. Initial experiments in chickens had shown that blue light, moving the plane of focus more anteriorly and in front of the retina, also inhibits eye growth, while red light, associated with a longer focal length, made eyes grow longer 11 , 12 . Eye growth seemed to follow the focal plane position imposed by longitudinal chromatic aberrations (LCA). Results became the more complicated, the more animal models were studied. In particular, in tree shrews and monkeys, red light made the eyes shorter rather than longer 13 , 14 . Blue or near ultraviolet reduced myopia development that was induced by negative lenses in mice 15 , as well as myopia induced by frosted eye occluders in chickens 16 . Also, human eyes became transiently shorter in narrowband blue light and longer in narrowband green and red light 17 . These findings suggest that the retinal mechanisms for the control of eye growth need “reference points” across the entire visible spectrum. But how could the retina combine the information derived from the full visible spectrum? While it is possible that this could be done by comparing irradiances at different wavelengths and their temporal patterns 18 , it would be more intuitive that image focus at different wavelengths is compared. The idea is not new and has been discussed by others before 19 , 20 . Already Fincham in 1951 assumed that accommodation, the lenticular mechanism to focus the eye at different viewing distances, uses chromatic differences in focal planes 21 .
To find out whether the retina can indeed compare focus at different wavelengths, we filtered conventional movies in real time, using custom-developed software in Visual C++. Each pixel in the movies was convolved with the point spread function calculated from purely spherical defocus that originated from the human LCA function separately in the red, green, and blue image plane of RGB channels (see “ Methods ”) 22 . The calculated chromatic aberration function was either added to the natural chromatic aberration in the subject’s eyes or was presented inverted so that the total chromatic aberration function became flat at the cost of some blur in all channels (see “ Methods ”). In this case, the retina perceived similar blur at both ends of the spectrum.
The hypothesis was that red in better focus than blue would indicate to the retina that the eye is already too long, and that eye growth should be inhibited. Conversely, if blue is presented in better focus than red, it was expected that eye growth would increase. Of course, an important control experiment is that subjects also watched unfiltered movies with only their natural LCA. In the current experiment, as well as in many other studies, axial length is defined as the distance from the corneal apex to the vitreo-retinal interface. This distance can be measured with high repeatability using optical low coherence interferometry. If short-term changes occur, they trace back to changes in choroidal thickness. Therefore, the presented effects reflect changes in choroidal thickness. As in previous studies, the induced changes in the length of the eyes were tracked using low coherence interferometry, the Lenstar LS 900 (Haag Streit, Koenitz, Switzerland) 23 , 24 . It had been confirmed by others that the small changes in axial length trace back to changes in choroidal thickness (i.e. 25 ) which, in turn, can predict future changes in eye growth and myopia development (i.e. 26 ). Also in the current study, no changes were observed in corneal thickness (CT) or anterior chamber depth (ACD) in any of the experiments.
Results are shown in Fig. 1 . Repeated-measures ANOVA revealed a significant influence of presented filters on change in axial length over time (F = 19.09, df = 2, p < 0.0001). With image focused more in red, occurred significant axial eye shortening over time (repeated-measures ANOVA F = 4.42, p = 0.007), as predicted by the hypotheses raised above (“red in focus” filter two-sided pairwise comparison: change in axial length after 45 min compared with a baseline: − 11.2 ± 14.1 μm, p = 0.013 (red lines); compared to control condition with unfiltered movies − 11.2 ± 14.1 μm vs. control − 2.2 ± 8.2 μm, two-sided pairwise comparison t = 2.42, df = 30, p = 0.01 (black lines)). Strikingly, no such changes were observed in myopic eyes (change after 45 min: + 7.1 ± 16.0 μm, two-sided pairwise comparison n.s., two-sided unpaired comparison to emmetropes: p = 0.001).
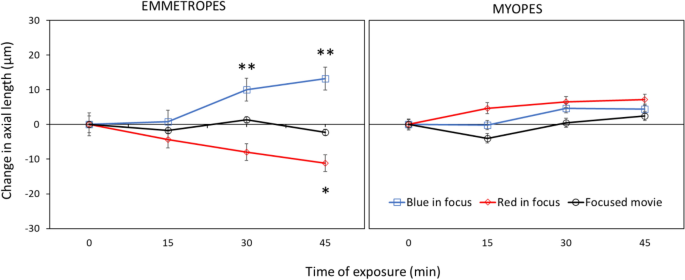
Changes in ocular axial length when subjects watched movies that were digitally filtered to present the red (“red in focus”) or blue (“blue in focus”) image plane in best focus. In emmetropic eyes, “red in focus” caused significant eye shortening (repeated measures ANOVA: p = 0.007) while “blue in focus” caused longer eyes (p = 0.0001). Strikingly, myopic eyes did not respond to these stimuli, indicating that they no longer detected the sign of defocus. Watching movies without chromatic filters did not induce changes in axial length, neither in emmetropes nor myopes (black lines). Data represents the averaged effect of all emmetropic (n = 20) and myopic (n = 15) participants. Error bars denote SEMs.
With calculated blur circles creating images more focus in blue and added to the natural LCA, the retinal image blur was imposed in both red and blue which induced significant eye elongation over time in emmetropes (repeated-measures ANOVA, F = 8.08, p < 0.001). Here, significant axial eye elongation was observed already after 30 min of stimulation (“blue in focus” filter two-sided pairwise comparison to the baseline: change in axial length after 30 min: + 10.0 ± 11.5 μm), and after 45 min (+ 13.2 ± 14.9 μm, both p < 0.01, blue lines). Axial eye length was also significantly increased compared to watching the unfiltered control movie (after 30 min: filter + 10.0 ± 11.5 μm vs. control + 1.4 ± 13.0 μm, two-sided pairwise comparison, t = 2.16, df = 35, p = 0.03; change after 45 min: filter + 13.2 ± 14.9 μm vs. control − 2.2 ± 8.2 μm, t = 4.01, df = 29, p = 0.0003). Moreover, there was a significant difference between change in axial length after watching “blue in focus” and “red in focus” movies after 45 min (+ 13.2 ± 14.9 μm vs. − 11.2 ± 14.1 μm, respectively, two-sided pairwise comparison, t = 5.29, df = 37, p < 0.0001).
Again, no changes were observed in myopic eyes (change in AL after 45 min: + 4.44 ± 18.1 μm, pairwise two-sided comparison, n.s.).
Neither emmetropes nor myopes showed any changes in eye length when they watched the unfiltered control movies (black lines; change in AL after 45 min: emmetropes − 2.2 ± 8.2 μm, myopes + 2.4 ± 13.0 μm, both two-sided pairwise comparison n.s.).
There was no influence of the time of day when the experiment was performed (morning or afternoon) or sex on changes in axial length in any part of the study (repeated-measures ANOVA with two within-subject factors: time of a day: p = 0.31, sex: p = 0.87, n.s.).
An important question is now: why does the myopic retina no longer respond to chromatic defocus? A possible explanation could be that contrast sensitivity in the blue is reduced in myopic eyes to an extent that the retina can no longer determine the level of defocus. The change cannot be detected in the green or red because mid- and long wavelength sensitive photoreceptors mediate high acuity, and it is known that moderately myopic subjects have normal visual acuity and contrast sensitivity 27 . Software was developed in Visual C++ to measure contrast sensitivity in the blue, green and red RGB channel, using a 4-FAC procedure. Circular spatial patterns with a fundamental spatial frequency around 3–5 cyc/deg were presented on a gray background. Subjects had to select in which quadrant they saw parts of the circular pattern, using the arrow keys on the keyboard. Thresholds were automatically determined by the software using a staircase procedure (see “ Methods ”). All participants, including 20 emmetropes and 15 myopes, were tested (Fig. 2 ). Both emmetropic and myopic subjects needed higher contrast to detect the pattern in the blue (emmetropes vs. myopes: 2.92 ± 0.93 vs. 3.11 ± 1.07 percent Weber contrast, respectively, n.s.), compared to green (emmetropes vs. myopes: 1.16 ± 0.38 vs. 1.14 ± 0.25 percent Weber contrast, respectively, n.s.) and red (emmetropes vs. myopes: 1.66 ± 0.59 vs. 1.64 ± 0.37, respectively, n.s.). Therefore, we did not find loss in contrast sensitivity in the blue in myopic subjects that could explain why their eyes did not respond to chromatic defocus.
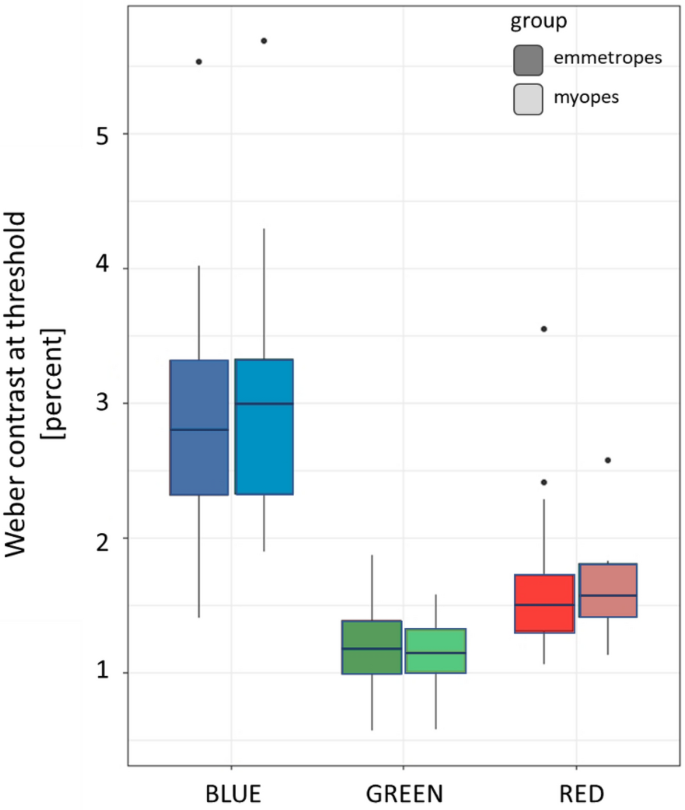
Weber contrast at the detection threshold in the blue, green, and red channel of the RGB format on the screen in emmetropic and myopic participants. No differences were observed between emmetropes and myopes.
The question how the retina can detect the sign of defocus has a long history and a number of different theories were developed over time: (1) a role of peripheral astigmatism which is high in human eyes but low in chicken eyes 28 , (2) a role of high order aberrations which can generate asymmetrical changes in the image when defocus is imposed with different sign 6 , (3) a role of the Stiles-Crawford effect which may change the signal strength when oblique rays are reaching the photoreceptors 29 (although a change in vergence resulting from 3 D of defocus changes the detected brightness by only less than a percent, and no sign of defocus information is generated), and finally (4) signals derived from longitudinal chromatic aberrations. The longitudinal chromatic aberrations appear most reliable because they contain information on the sign of defocus, are sufficiently large and require comparison of the output of only two photoreceptor types as found in most mammals (most are dichromats). The spatial resolution of blue cones in humans is about 5 cyc/deg, sufficient to detect a defocus of 1–2 D as needed for the mechanism 30 .
Because the blue image on the retina is already blurred by the natural LCA in the eye, we could not restore image sharpness in the blue by spatially filtering movies 31 . However, the red image could be blurred to a similar amount as the blue, so that the retina experienced similar blur in both spectral ranges (“blue in focus” filter), which we found to cause elongation of the eye. While blurry images are known to induce deprivation myopia, it is unlikely that this was the case here because images in the green were still reasonably well focused 32 . There must be a more specific mechanism behind—probably a comparison of sharpness in blue and red. We excluded in our study that loss of contrast sensitivity in the blue was responsible for the lacking response in myopes. An earlier study by Taylor et al. had found a minor decrease in contrast sensitivity in myopic subjects but the effects sizes were extremely small (p < 0.047 if one outlier of 44 observers was omitted) 33 . Since the differences between emmetropes and myopes in our study were prominent (Fig. 1 ), the findings by Taylor et al., are unlikely to explain the difference that we found between myopes and emmetropes. Therefore, other changes must have occurred in the retinal circuitry to compare the focus in the blue and red which need further studies.
After Rucker and Wallman 34 had concluded from their experiments in chickens that “although previous work has shown that chromatic cues to defocus are not essential for lens-compensation, in that chicks can compensate in monochromatic light, our evidence implies that the eye may be able to infer whether the eye is myopic or hyperopic from the different chromatic contrasts that result from different signs of defocus.” Later, Gawne et al. analyzed whether S cone density in primates may be high enough to detect defocus in the blue that was imposed from longitudinal chromatic aberration 35 . They concluded that “the retinal spacing of the short-wavelength sensitive cones in many mammalian species is an evolutionarily ancient adaptation that allows the efficient use of chromatic cues in emmetropization.” A little later, Gawne et al. showed that this approach actually worked in tree shrews 36 . Tree shrews could be made more hyperopic simply by covering just one wall of their cages with an RGB screen that displayed a black and white pattern that was heavily low pass filtered in the blue channel. As a result, black-white edges had yellowish color fringes. The striking result of this experiment agreed with the hypothesis that, if blue is out of focus (and low pass filtered), it should tell the retina that the eye is already too long and further growth should be inhibited—and this is exactly what they found. Our current study is different in three regards (1) it is in humans (2) it involves movies instead of stationary patterns and (3) we simulated the chromatic blur exactly according to the human chromatic aberration function. It may be that the blur in the blue was less than in the study by Gawne et al. 36 , and in fact, the differently filtered movies did not appear very different to the subjects but still had the described effects on choroidal thickness.
Recently, it was found in tree shrews that low pass filtering of the blue image, induced more hyperopic refractions 36 . In this study, animals were kept in narrow cages which induced some degree of myopia based on their short viewing distances. Myopia could be prevented if one wall of the cage was covered with artificial visual targets that were low pass filtered only in the blue. Their findings relate to our findings in humans, although interactions with natural LCA were not analyzed. Gawne and Norton also developed a model how the tree shrews emmetropize based on a comparison of image focus in the S- and M-cone plane, which supports the hypothesis that LCA controls emmetropization 19 .
Our study relied on the assumption that participants were focused in the green, as described by Wyszecki and Stiles 37 , Benedi and Garcia et al. 31 , and Marcos et al. 22 . However, accommodation was not directly measured. But even if accommodation would have not focused perfectly on the mid-wavelength range, a number of recent studies have shown that defocus imposed by inaccurate accommodation has no effect on emmetropization (chicken: 38 ; children: 39 , 40 ).
Using LCA as a signal for emmetropization imposes interesting limitations. In human eyes, chromatic aberration spans over a dioptric range of maximally 2.5 D. If an eye becomes myopic, it may move out of the range where a comparison of sharpness in the blue and red provides a useful signal, because both may then be out of focus. While this could explain why myopia tends to progress when it is out of regulated range, it does not explain why optical correction does not restore the mechanism—and why refractions initially move out of the regulated range at all.
Nature rarely relies on one simple mechanism to control an important variable (in this case, refraction). Looking back at myopia research in the past, it becomes clear that several previous descriptions of “the mechanism of myopia” fell all short and described only one aspect of a large picture. Therefore, the current findings may also reflect only a part of emmetropization. Nevertheless, we still believe that our findings of functional deficiencies in the myopic retina move the question of myopia progression to another level. We are aware that there are several optical treatments that can reduce myopia progression, despite that we found that the myopic retina has reduced ability to detect positive defocus 23 . But is it really safe to assume that successful optical treatments (like DIMS designs or multifocal designs) work because they impose positive defocus on the retina? It could be any other kind of stimulation. Why should retinal image diffusing lens designs have any effect? They do not impose positive defocus. On the other hand, in our experiments we had a very few myopic subjects who responded to positive defocus or chromatic filtering just as emmetropes. A possible explanation is that their myopia was stable since long and that the emmetropization mechanism had partially recovered, keeping their refraction at an optimum with the optical correction in place.
Conclusions
Our results demonstrate for the first time that the human retina uses the difference in focus in the blue and the red to determine the sign of defocus for emmetropization. Strikingly, this function is lost in myopes. While we had previously found that the myopic human retina has limited ability to respond to imposed positive defocus, the current results show now that the myopic retina has lost the ability to respond to longitudinal chromatic aberration.
Thirty-five young adult participants (average age: 26 ± 3 years) were recruited. Prior to the experiments spherical equivalents of right eyes were measured without cycloplegia using a commercially available infrared photorefractor (PlusoptiX A12R binocular autorefractor, PlusOptix, Nuremberg, Germany). The experimental group included 20 emmetropes (7 males) with an average refractive error of − 0.38 ± 0.44 D and 15 myopes (5 males) with an average refractive error of − 3.41 ± 1.25 D. Subjects with astigmatism larger than 1D were excluded from the study. None of the subjects suffered from ocular pathologies other than moderate refractive errors. Informed consent was signed by each of the participants prior to the experiments. The study was approved by the Swiss Research Ethics Commission (EKNZ, reference 2020-01576) and met the requirements of the Declaration of Helsinki.
The participants were asked to watch binocularly a movie on a large TV screen (65 inches, LG OLED65C9, 4 K, 2019) at 2 m distance in a dark room. Myopic subjects wore their habitual corrections. The experiment was performed between 8 AM and 4 PM. The study protocol included 3 appointments on 3 separate days at the same time of the day for each individual participant. Day 1: watching the unfiltered movie served as a control, day 2: watching the movie digitally filtered to present the blue image sharp, but green and red low pass filtered according to the LCA function, and day 3: watching the movie digitally filter to present the red image sharp, and green and blue filtered according to the LCA function. Changes in axial length of the right eyes were measured during each session, before and after 15, 30 and 45 min of watching a movie. To avoid any additional factors that could influence the results, the same part of the movie was played for all conditions and for all participants. Moreover, the participants were asked to refrain from drinking coffee or smoking two hours prior to the experiment.
After the control session, each participant performed a contrast sensitivity test for red, green, and blue spatial stimuli, displayed on gray background.
Ocular biometry
Axial length (measured as the distance between the outer surface of the cornea and RPE), corneal thickness (CT) and anterior chamber depth (ACD) of the right eyes were assessed by using a low coherence interferometer with auto positioning system (Lenstar LS 900 Haag-Streit, Koeniz, Switzerland). Measurements were done before and immediately after every 15 min of watching chromatically filtered or the control movies. The average data from three repeated measurements were used. Due to the auto positioning system of the device and an extensively trained operator, standard deviations were typically around 5 µm for the axial length measurements.
Chromatic spatial filtering
Movies were chromatically filtered in real-time by custom-developed software written in Visual C++, which allowed to individually blur each of the RGB channels (red, green, or blue). With a video format of 1280 × 720 pixels, filtering was possible at about 25 Hz (Hz) frame rate. To apply the same frame rates to all experimental conditions, also the unfiltered control movie was run through the software. Spatial filtering had no effect on the average pixel values in the three RGB channels which were continuously shown by the software. Therefore, the appearance of color of the movies did not change during filtering. Two chromatic filters were used: “red in focus” where red pixels were untouched, while green and blue pixels were blurred (Fig. 3 ), and “blue in focus” where blue pixels were untouched while green and red pixels were blurred (Fig. 3 ). The combination of blur circle generated by natural LCA (Fig. 3 , box 1) and calculated LCA (Fig. 3 , box 2) let to blur circles on the retina that either caused low pass filtering in the blue and a sharp image in the red (filter “red in focus”) or low pass filtering in the red, but combined with LCA blur in the blue (filter “blue in focus”) (Fig. 3 , box 3). The important difference was that once red appeared sharp and blue blurry (“red in focus”), and once both appeared blurry (“blue in focus”). If the retina performs a comparison of sharpness in blue and red, the induced changes in axial eye length should be different in both cases. For the calculations of the blur circles, a pupil size of 6.5 mm was used which was the average pupil size of all subjects. There was no difference between emmetropic and myopic subjects in our sample. The calculations assumed that subjects were in best focus at 570 nm as proposed by Marcos et al. 22 . The calculated blur circles diameters can be readily converted into angular units. In the human retina, 1 deg in the visual field maps to 290 µm on the retina. Accordingly, a blur circle diameter of 14.3 mm at 2000 mm distance corresponds to 24.6 arcmin, using that 1 pixel on our large TV screen had a diameter of 1.13 mm, equivalent to 1.94 arcmin.
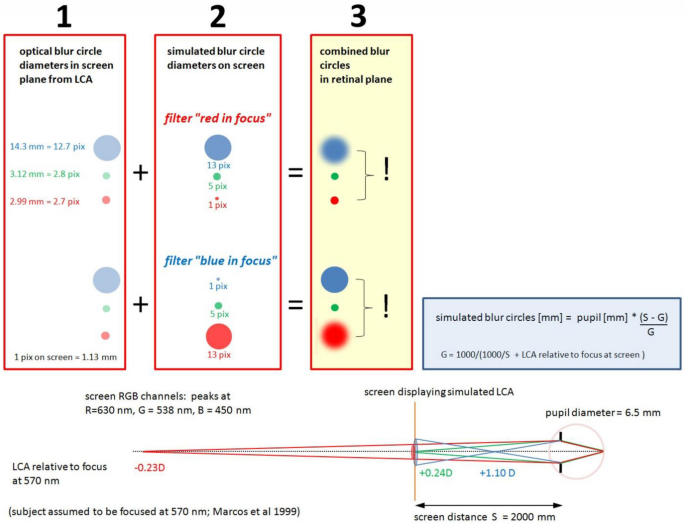
Subjects watched movies on the screen at 2000 mm distance. As proposed by Marcos et al. 22 , subjects are assumed to be in best focus at 570 nm (see illustration at the bottom of the Figure). According to the LCA function in human subjects (shown below in Fig. 4 ), the red channel in RGB (630 nm) is defocused hyperopically by − 0.23D, the blue channel (450 nm) myopically defocused by + 1.10D while the RGB green channel (538 nm) is slightly myopically defocused by + 0.24D. The respective calculated blur circles are shown in red box 1. Movies were filtered as shown in red box 2. With the filter “red in focus”, the image of the red channel remained untouched while green and blue were spatially filtered according to the LCA. With the filter “blue in focus”, the image of the blue channel remained untouched while red and green were spatially filtered according to the LCA. Combining box 1 and 2, the blur circles on the retina could be changed so that either blue was low pass filtered and red was sharp, or both were low pass filtered by combining natural LCA with the calculated blur circles accordingly to the LCA function (box 3).
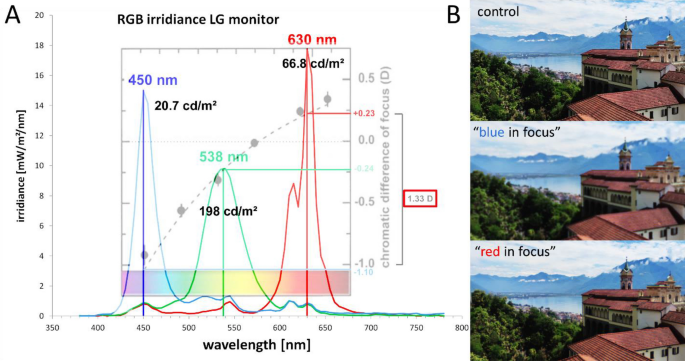
( A ) Spectral irradiances of the blue, green and red channel on the RGB screen, and the respective peak wavelengths, as measured with a commercial photospectrometer (Gossen, MAVOSPEC BASE, Nuremberg, Germany). The dotted line shows the chromatic defocus function, relative to 570 nm, as provided by Marcos et al. 22 . Black numbers indicate the luminance, separately measured with a Minolta candela meter (LS-100, Minolta Camera Co., LTD, Tokyo, Japan). Point spread functions for the chromatic blur were calculated relative to 570 nm (Fig. 3 ). ( B ) Illustration of images filtered with the “blue in focus” and “red in focus” filter. Note that image with “blue in focus” filter appears more blurry than the image with “red in focus” filter because the human visual system uses M and L cones for high acuity tasks and blur in red and green has higher impact of visual acuity.
The human chromatic aberration function and the derived chromatic defocus used to calculate the blur circle diameters in Fig. 3 is shown in Fig. 4 (after Marcos et al. 22 ).
The modulation transfer functions in red, green, and blue were also determined. We used a RGB camera (DFK 33UX290, Imaging Source, Germany, 1920 × 1080 px resolution) with similar optical parameters as the human eye with 6.5 mm pupil (16 mm focal length, f/# 2.6) and 16 mm lens (M1614-MP2, Computar, Japan) to obtain spatial frequency spectra for control, “blue in focus” and “red in focus” movies. Pictures of the three conditions were taken from the screen showing the movies at 2 m distance. RGB channels were separated, and Fourier analysis performed with publicly available software (ImageJ, National Institutes of Health [NIH], http://imagej.nih.gov/ij/ ). Subsequently, one-dimensional plots were created by rotational averaging of the two-dimensional spatial frequency spectra, using custom-developed software written in Visual C++. Averaged pixel values were normalized to the maximum of 1 and the minimum of 0 for each RGB channel in every experimental condition, as shown in Fig. 5 .
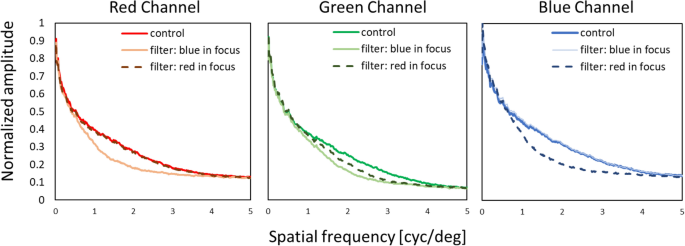
Spatial frequency spectra determined by Fourier analysis of the control, “blue in focus” and “red in focus” filtering condition.
Measurement of contrast sensitivity in RGB red, green, and blue
Custom-developed software written in Visual C++ was used to display annular hemi-circular patterns of dots of varying size on a grey screen (RGB (127,127,127) either in red, green, or blue (DELL U2518D, 25″, 1920 × 1080 px resolution) at 50 cm distance. Chromatic hue was generated by simply increasing the individual RGB values of the dots in steps of one (i.e., red RGB (128,127,127). The pattern appeared randomly on the top, right, down, or left side. Participants had to indicate where they see the pattern using the arrow keys (up, down, right, or left) of the keyboard. If the answer was correct, the pixel brightness value in the tested channel was reduced by 1, moving its color closer to grey. When subjects made wrong choice, pattern contrast was increased by 3-pixel values and then reduced again when the selection was correct. After 25 selections, the lowest contrast of 5 correct choices were averaged and used as a measure of the detection threshold. Contrast of the pattern was measured as Weber fraction after an initial individual calibration of the screen luminance in R, G, and B with a Minolta candela meter. Background luminance of the screen was 49.64 cd/m 2 and starting Weber contrast was about 10% for all color patterns. Results from three repeated tests were averaged and served as a threshold value of Weber contrast for each individual participant, separately for each color (Fig. 6 ).
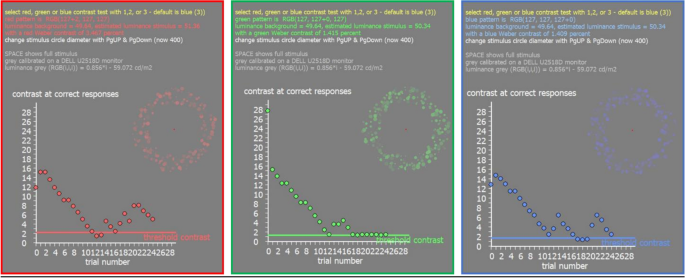
Illustration of the screen output of the software to measure contrast sensitivity in R, G, and B. In the top right of each picture, the appearance of the stimuli is illustrated but during the real test, they cover a major part of the screen (diameter of the circular pattern 400 pixel). In the bottom, the output of the staircase procedure is shown.
Statistical analyses
All statistical calculations were performed using the publicly available software environment R (version R 4.1.0; R Core Team, R Foundation for Statistical Computing, Vienna, Austria). Data from each refractive group are presented as the averages from either all myopic or emmetropic subjects, together with the standard errors. The QQ plots were used to confirm that the axial length data were normally distributed. Changes in axial lengths during watching digitally filtered or control movies were analyzed using a repeated measures analysis of variance (ANOVA) with two within-subjects factors of time and type of filter (“blue in focus”, “red in focus”, control), followed by a post hoc test with Bonferroni correction. In addition, influence of two between-subjects factors (time of day when experiment was performed: morning or afternoon, and sex) was calculated for each tested condition. Changes in CT + ACD before and after 45 min of stimulation were analyzed using a paired Student’s T-test. The results of the contrast sensitivity measurements were analyzed separately for blue, green, and red by using Wilcoxon test to compare differences between myopic and emmetropic eyes.
Data availability
The datasets used and/or analysed during the current study available from the corresponding author on reasonable request (Dr. Barbara Swiatczak [email protected]). The software to simulate LCA in real time in images provided by a (laptop) camera can be downloaded here (exe file and libraries only) https://www.dropbox.com/sh/u1ppxub1ef3exnp/AAAH2NwA03vc7T2NpoWf9l4ma?dl=0 (after starting the “RGB LCA with text.exe” first select your camera and select MJPEG(1280x720) video format). The software to measure contrast sensitivity in R, G and B can be downloaded here (exe file only): https://www.dropbox.com/s/qy2xejy5p9zb256/blue%20cone%20contrast.exe?dl=0 .
Holden, B. A. et al. Global prevalence of myopia and high myopia and temporal trends from 2000 through 2050. Ophthalmology 123 , 1036–1042. https://doi.org/10.1016/j.ophtha.2016.01.006 (2016).
Article Google Scholar
Ohno-Matsui, K. et al. IMI pathologic myopia. Invest. Ophthalmol. Vis. Sci. 62 , 5. https://doi.org/10.1167/iovs.62.5.5 (2021).
Morgan, I. & Rose, K. How genetic is school myopia?. Prog. Retin. Eye Res. 24 , 1–38. https://doi.org/10.1016/j.preteyeres.2004.06.004 (2005).
Gajjar, S. & Ostrin, L. A. A systematic review of near work and myopia: Measurement, relationships, mechanisms and clinical corollaries. Acta Ophthalmol. 100 , 376–387. https://doi.org/10.1111/aos.15043 (2022).
Troilo, D. & Wallman, J. The regulation of eye growth and refractive state: An experimental study of emmetropization. Vision Res. 31 , 1237–1250. https://doi.org/10.1016/0042-6989(91)90048-a (1991).
Article CAS Google Scholar
Wallman, J. & Winawer, J. Homeostasis of eye growth and the question of myopia. Neuron 43 , 447–468. https://doi.org/10.1016/j.neuron.2004.08.008 (2004).
Schaeffel, F. & Diether, S. The growing eye: An autofocus system that works on very poor images. Vision Res. 39 , 1585–1589. https://doi.org/10.1016/s0042-6989(98)00304-6 (1999).
Zhu, X., Winawer, J. A. & Wallman, J. Potency of myopic defocus in spectacle lens compensation. Invest. Ophthalmol. Vis. Sci. 44 , 2818–2827. https://doi.org/10.1167/iovs.02-0606 (2003).
French, A. N., Morgan, I. G., Mitchell, P. & Rose, K. A. Risk factors for incident myopia in Australian schoolchildren: The Sydney adolescent vascular and eye study. Ophthalmology 120 , 2100–2108. https://doi.org/10.1016/j.ophtha.2013.02.035 (2013).
He, X. et al. Time outdoors in reducing myopia: A school-based cluster randomized trial with objective monitoring of outdoor time and light intensity. Ophthalmology https://doi.org/10.1016/j.ophtha.2022.06.024 (2022).
Seidemann, A. & Schaeffel, F. Effects of longitudinal chromatic aberration on accommodation and emmetropization. Vision Res. 42 , 2409–2417. https://doi.org/10.1016/s0042-6989(02)00262-6 (2002).
Foulds, W. S., Barathi, V. A. & Luu, C. D. Progressive myopia or hyperopia can be induced in chicks and reversed by manipulation of the chromaticity of ambient light. Invest Ophthalmol. Vis. Sci. 54 , 8004–8012. https://doi.org/10.1167/iovs.13-12476 (2013).
Gawne, T. J., Ward, A. H. & Norton, T. T. Long-wavelength (red) light produces hyperopia in juvenile and adolescent tree shrews. Vision Res. 140 , 55–65. https://doi.org/10.1016/j.visres.2017.07.011 (2017).
Hung, L. F., Arumugam, B., She, Z., Ostrin, L. & Smith, E. L. 3rd. Narrow-band, long-wavelength lighting promotes hyperopia and retards vision-induced myopia in infant rhesus monkeys. Exp. Eye Res. 176 , 147–160. https://doi.org/10.1016/j.exer.2018.07.004 (2018).
Jiang, X. et al. Violet light suppresses lens-induced myopia via neuropsin (OPN5) in mice. Proc. Natl. Acad. Sci. USA 118 , 5236. https://doi.org/10.1073/pnas.2018840118 (2021).
Wang, M., Schaeffel, F., Jiang, B. & Feldkaemper, M. Effects of light of different spectral composition on refractive development and retinal dopamine in chicks. Invest. Ophthalmol. Vis. Sci. 59 , 4413–4424. https://doi.org/10.1167/iovs.18-23880 (2018).
Thakur, S., Dhakal, R. & Verkicharla, P. K. Short-term exposure to blue light shows an inhibitory effect on axial elongation in human eyes independent of defocus. Invest Ophthalmol. Vis. Sci. 62 , 22. https://doi.org/10.1167/iovs.62.15.22 (2021).
Watts, N. S., Taylor, C. & Rucker, F. J. Temporal color contrast guides emmetropization in chick. Exp. Eye Res. 202 , 108331. https://doi.org/10.1016/j.exer.2020.108331 (2021).
Gawne, T. J. & Norton, T. T. An opponent dual-detector spectral drive model of emmetropization. Vision Res. 173 , 7–20. https://doi.org/10.1016/j.visres.2020.03.011 (2020).
Troilo, D. et al. IMI—report on experimental models of emmetropization and myopia. Invest Ophthalmol. Vis. Sci. 60 , M31–M88. https://doi.org/10.1167/iovs.18-25967 (2019).
Fincham, E. F. The accommodation reflex and its stimulus. Br. J. Ophthalmol. 35 , 381–393. https://doi.org/10.1136/bjo.35.7.381 (1951).
Marcos, S., Burns, S. A., Moreno-Barriusop, E. & Navarro, R. A new approach to the study of ocular chromatic aberrations. Vision Res. 39 , 4309–4323. https://doi.org/10.1016/s0042-6989(99)00145-5 (1999).
Swiatczak, B. & Schaeffel, F. Emmetropic, but not myopic human eyes distinguish positive defocus from calculated blur. Invest. Ophthalmol. Vis. Sci. 62 , 14. https://doi.org/10.1167/iovs.62.3.14 (2021).
Read, S. A., Collins, M. J. & Sander, B. P. Human optical axial length and defocus. Invest. Ophthalmol. Vis. Sci. 51 , 6262–6269. https://doi.org/10.1167/iovs.10-5457 (2010).
Chakraborty, R. et al. Effects of mild- and moderate-intensity illumination on axial length and choroidal thickness in young adults. Invest. Ophthalmol. Vis. Sci. 62 , 1336–1336 (2021).
Google Scholar
Read, S. A., Alonso-Caneiro, D., Vincent, S. J. & Collins, M. J. Longitudinal changes in choroidal thickness and eye growth in childhood. Invest. Ophthalmol. Vis. Sci. 56 , 3103–3112. https://doi.org/10.1167/iovs.15-16446 (2015).
Liou, S. W. & Chiu, C. J. Myopia and contrast sensitivity function. Curr. Eye Res. 22 , 81–84. https://doi.org/10.1076/ceyr.22.2.81.5530 (2001).
Maier, F. M., Howland, H. C., Ohlendorf, A., Wahl, S. & Schaeffel, F. Lack of oblique astigmatism in the chicken eye. Vision Res. 109 , 68–76. https://doi.org/10.1016/j.visres.2015.02.002 (2015).
Vohnsen, B. Geometrical scaling of the developing eye and photoreceptors and a possible relation to emmetropization and myopia. Vision Res. 189 , 46–53. https://doi.org/10.1016/j.visres.2021.09.002 (2021).
Calkins, D. J. Seeing with S cones. Prog. Retin Eye Res. 20 , 255–287. https://doi.org/10.1016/s1350-9462(00)00026-4 (2001).
Benedi-Garcia, C. et al. Vision is protected against blue defocus. Sci. Rep. 11 , 352. https://doi.org/10.1038/s41598-020-79911-w (2021).
Wallman, J., Turkel, J. & Trachtman, J. Extreme myopia produced by modest change in early visual experience. Science 201 , 1249–1251. https://doi.org/10.1126/science.694514 (1978).
Article ADS CAS Google Scholar
Taylor, C. P., Shepard, T. G., Rucker, F. J. & Eskew, R. T. Jr. Sensitivity to S-cone stimuli and the development of myopia. Invest. Ophthalmol. Vis. Sci. 59 , 4622–4630. https://doi.org/10.1167/iovs.18-24113 (2018).
Rucker, F. J. & Wallman, J. Chick eyes compensate for chromatic simulations of hyperopic and myopic defocus: Evidence that the eye uses longitudinal chromatic aberration to guide eye-growth. Vision Res. 49 , 1775–1783. https://doi.org/10.1016/j.visres.2009.04.014 (2009).
Gawne, T. J., Grytz, R. & Norton, T. T. How chromatic cues can guide human eye growth to achieve good focus. J. Vis. 21 , 11. https://doi.org/10.1167/jov.21.5.11 (2021).
Gawne, T. J., She, Z. & Norton, T. T. Chromatically simulated myopic blur counteracts a myopiagenic environment. Exp. Eye Res. 222 , 109187. https://doi.org/10.1016/j.exer.2022.109187 (2022).
Wyszecki, G. & Stiles, W. S. Color Science: Concepts and Methods, Quantitative Data and Formulae (Wiley, 2000).
Aleman, A. & Schaeffel, F. Lag of accommodation does not predict changes in eye growth in chickens. Vision Res. 149 , 77–85. https://doi.org/10.1016/j.visres.2018.06.007 (2018).
Chen, Y. et al. Accommodation is unrelated to myopia progression in Chinese myopic children. Sci. Rep. 10 , 12056. https://doi.org/10.1038/s41598-020-68859-6 (2020).
Lan, W., Yang, Z., Liu, W., Chen, X. & Ge, J. A longitudinal study on the relationship between myopia development and near accommodation lag in myopic children. Ophthalmic Physiol. Opt. 28 (1), 57–61. https://doi.org/10.1111/j.1475-1313.2007.00536.x (2008).
Download references
Author information
Authors and affiliations.
Institute of Molecular and Clinical Ophthalmology Basel (IOB), Basel, Switzerland
Barbara Swiatczak & Frank Schaeffel
Section of Neurobiology of the Eye, Ophthalmic Research Institute, University of Tuebingen, Tuebingen, Germany
Frank Schaeffel
Zeiss Vision Lab, Ophthalmic Research Institute, University of Tuebingen, Tuebingen, Germany
You can also search for this author in PubMed Google Scholar
Contributions
Both authors contributed equally to this work.
Corresponding author
Correspondence to Barbara Swiatczak .
Ethics declarations
Competing interests.
The authors declare no competing interests.
Additional information
Publisher's note.
Springer Nature remains neutral with regard to jurisdictional claims in published maps and institutional affiliations.
Rights and permissions
Open Access This article is licensed under a Creative Commons Attribution 4.0 International License, which permits use, sharing, adaptation, distribution and reproduction in any medium or format, as long as you give appropriate credit to the original author(s) and the source, provide a link to the Creative Commons licence, and indicate if changes were made. The images or other third party material in this article are included in the article's Creative Commons licence, unless indicated otherwise in a credit line to the material. If material is not included in the article's Creative Commons licence and your intended use is not permitted by statutory regulation or exceeds the permitted use, you will need to obtain permission directly from the copyright holder. To view a copy of this licence, visit http://creativecommons.org/licenses/by/4.0/ .
Reprints and permissions
About this article
Cite this article.
Swiatczak, B., Schaeffel, F. Myopia: why the retina stops inhibiting eye growth. Sci Rep 12 , 21704 (2022). https://doi.org/10.1038/s41598-022-26323-7
Download citation
Received : 29 August 2022
Accepted : 13 December 2022
Published : 15 December 2022
DOI : https://doi.org/10.1038/s41598-022-26323-7
Share this article
Anyone you share the following link with will be able to read this content:
Sorry, a shareable link is not currently available for this article.
Provided by the Springer Nature SharedIt content-sharing initiative
By submitting a comment you agree to abide by our Terms and Community Guidelines . If you find something abusive or that does not comply with our terms or guidelines please flag it as inappropriate.
Quick links
- Explore articles by subject
- Guide to authors
- Editorial policies
Sign up for the Nature Briefing: Translational Research newsletter — top stories in biotechnology, drug discovery and pharma.

ORIGINAL RESEARCH article
Global tendency and frontiers of research on myopia from 1900 to 2020: a bibliometrics analysis.
A correction has been applied to this article in:
Corrigendum: Global tendency and frontiers of research on myopia from 1900 to 2020: A bibliometrics analysis
- Read correction
- 1 School of Medicine, Nankai University, Tianjin, China
- 2 Clinical College of Ophthalmology, Tianjin Medical University, Tianjin, China
- 3 Tianjin Key Laboratory of Ophthalmology and Visual Science, Tianjin Eye Institute, Tianjin Eye Hospital, Nankai University Affiliated Eye Hospital, Tianjin, China
Background: Myopia is one of the most common causes of vision impairment in children and adults and has become a public health priority with its growing prevalence worldwide. This study aims to identify and evaluate the global trends in myopia research of the past century and visualize the frontiers using bibliometric analysis.
Methods: The literature search was conducted on the Web of Science for myopia studies published between 1900 and 2020. Retrieved publications were analyzed in-depth by the annual publication number, prolific countries and institutions, core author and journal, and the number of citations through descriptive statistics. Collaboration networks and keywords burst were visualized by VOSviewer and CiteSpace. Myopia citation network was visualized using CitNetExplorer.
Results: In total, 11,172 publications on myopia were retrieved from 1900 to 2020, with most published by the United States. Saw SM, from the National University of Singapore, contributed the most publications and citations. Investigative Ophthalmology & Visual Science was the journal with highest number of citations. Journal of Cataract and Refractive Surgery with the maximum number of publications. The top 10 cited papers mainly focused on the epidemiology of myopia. Previous research emphasized myopia-associated experimental animal models, while recent keywords include “SMILE” and “myopia control” with the stronger burst, indicating a shift of concern from etiology to therapy and coincided with the global increment of incidence. Document citation network was clustered into six groups: “prevalence and risk factors of myopia,” “surgical control of myopia,” “pathogenesis of myopia,” “optical interventions of myopia,” “myopia and glaucoma,” and “pathological myopia.”
Conclusions: Bibliometrics analysis in this study could help scholars comprehend global trends of myopia research frontiers better. Hundred years of myopia research were clustered into six groups, among which “prevalence and risk factors of myopia” and “surgical control of myopia” were the largest groups. With the increasing prevalence of myopia, interventions of myopia control are a potential research hotspot and pressing public health issue.
Introduction
Myopia, also known as short-sightedness or near-sightedness, is one of the most prevalent eye disorders worldwide that lead to vision impairment in young individuals ( 1 ). It is one of the five ocular conditions listed as an immediate priority by the World Health Organization's Global Initiative for the Elimination of Avoidable Blindness. A meta-analysis predicted that up to half of the world's population would have myopia by 2050, 10% of which would have high myopia ( 2 ). The recent findings around the world imply an increased myopia incidence and myopia progression during the COVID-19 pandemic. The increasing prevalence combined with the rising early onset of myopia, which naturally leads to an increased risk of high myopia ( 3 ). High myopia can generate irreversible blindness owing to the secondary changes in the choroid, retina, and sclera ( 4 ). Optical interventions, such as spectacles, contact lenses, and refractive surgeries can correct the refractive error; however, they may not prevent high myopia-related complications ( 5 ). The large number of patients suffering from myopia and its impact on public health, such as its economic burden and quality of life implications, makes a bibliometric analysis of research studies significant.
Since E.W. Hulme, a British library scientist, first put forward “Statistical Bibliography” in 1922, bibliometric analysis has continued for nearly a 100 years ( 6 ). The field started to attract widespread attention with the proliferation of easily accessible online databases and the development of analysis software. Bibliometric analysis is a method that gives a valuable overview of existing academic literature and predicts the development trends of research based on citation reports and content, using mathematical and statistical methods ( 7 ). To date, bibliometric analysis has been applied to explore the development and trends of a specific field ( 8 – 10 ).
The research on myopia is so extensive, the number of publications is enormous and the research directions are different which make it difficult to identify the research focus and frontiers in the field. Thus, the study aimed to manifest a general status of global myopia research based on Web of Science (WOS) data from the entire 20th century. The bibliometric method was applied to analyze the research focus, frontiers, and key publications of myopia combined with citation network, and explore the research trend by keywords burst, to provide a comprehensive and promising reference for interested researchers.
Materials and Methods
Sources of the data and search strategy.
The search for papers to be included in this study was carried out in July 2021 through the Web of Science Core Collection (WOS) provided by Thomson Reuters (Philadelphia, PA, USA). There are many databases available for worldwide research assessment; however, the WOS database is one of the most comprehensive databases with papers dating back to the year 1900 ( 11 ). We used the advanced feature and selected the keywords “myopia,” “nearsightedness,” or “shortsightedness” in the title and/or abstracts. The search strategy was as follows: TI = “Myopia” OR AB = “Myopia” OR TI=“nearsightedness” OR AB = “nearsightedness” OR TI = “shortsightedness” OR AB = “shortsightedness.” Only articles and reviews were included as the document types. There were no language restrictions for literature collection. The search covered the period from 1900 to 2020. Data were downloaded from WOS in “plain text” format with “full record and cited references.” The search strategy for the terms related to Myopia was restricted to Title/Abstract to achieve greater accuracy in the results because many reported publications were not related to Myopia if applied to other search fields such as keywords. The use of title/abstract search is recommended in the bibliometric studies in contrast to the title-abstract-keywords search query because it substantially increases the specificity with minimum loss of sensitivity.
Bibliometric Software
In bibliometric analysis, the annual number of publications, prolific countries and institutions, core author and journal, paper citations, keywords, and bibliometric indicators are presented through descriptive analysis. The built-in analysis tool of Web of Science can create the citation network, but it is limited to offering the connections that exist between the citations of specific groups of articles and the co-authorship between the specific items. We applied bibliometric software to this study due to this reason. CitNetExplorer software was used to evaluate the development of scientific research within a specific field, which enable the researcher to visualize the citation networks and the relationship among these articles ( 12 ). VOSviewer software offers text mining functionality that can be used to construct and visualize co-occurrence networks of important terms extracted from a body of scientific literature, represented as nodes and links ( 13 ). The nodes size represented the number, and the links between the nodes reflected the partnership between the items. The graphic display ability of CiteSpace is not as strong as that of the VOSviewer, but it has the unique burst analysis function of keywords, which can demonstrate the changes in the hot spots in this field ( 14 ).
Data Analysis
The data from the WOS database was imported to the bibliometric software to produce visualization results and quantitative analysis for researchers. For this analysis, the most common bibliometric indicators were used: the number of publications, the number of citations. Microsoft Excel was used to arrange and sort the data, and extract the top results. The publication citation network was calculated using CitNetExplorer software. The setting of the clustering parameters resolution was set at 1.20 and a minimum cluster size of 1,000 articles. The co-authorship networks of countries, authors, organizations were made by VOSviewer, respectively. We chose the optimized parameter, which is described in detail in each figure notes. The burst keywords were assessed using CiteSpace software with the following parameters: time slicing (1990–2020), years per slice ( 1 ), term source (title, abstract, author keyword, keyword plus), node type (keyword), selection criteria (top 30).
Description of Publication
Growth trends of publications.
Based on the WOS database analysis, 11 172 documents on myopia published between 1900 and 2020 were retrieved. The first article on myopia was published in 1907. Prior to 1990, this field of research had not received much attention. Since 1991, the number of articles published increased gradually from 100 publications to over 400 after 2011 ( Figure 1 ). There were 822 articles published in 2020. In 2021, 429 articles have been published as of June, and the number is likely to increase.
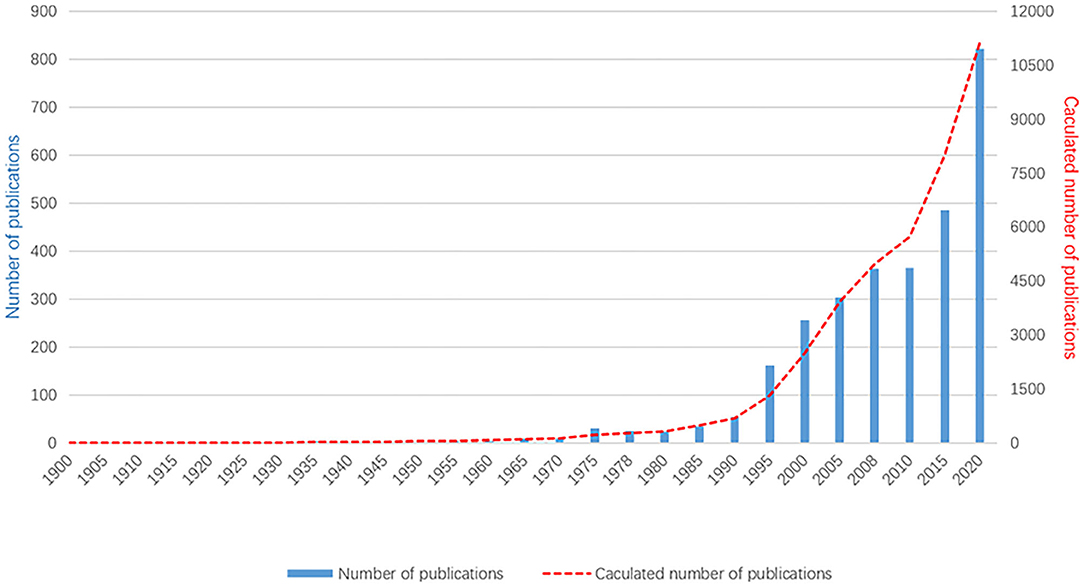
Figure 1 . The annual number of published myopia studies, 1900–2020. As the data in 2021 are still updated, they are not included in this figure.
Distribution of Countries
According to the retrieved articles, the articles on myopia originated from 127 countries. Table 1 shows that the United States accounted for the most number of articles published (19.82%), followed by China and Australia. Studies from the United States were cited 105 738 times, ranking first among all countries, followed by Australia and China. The collaboration relationship was analyzed using VOSviewer. As shown in Supplementary Figure 1 , the United States (USA), the largest node, is the most active country in this field. The cooperation map showed that the USA intensively collaborated with many countries in myopia fields, such as Germany, France, and Spain.
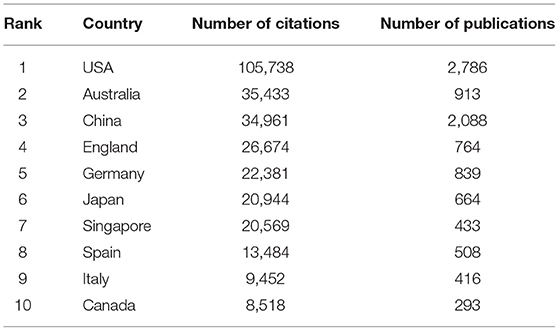
Table 1 . Top 10 most influential countries in myopia research.
Distribution of Authors
According to the retrieved results, over 71,292 authors contributed to myopia research. Table 2 lists the 10 most productive authors in the field of myopia research. Among all authors, Saw SM contributed the most publications (175), the most citations (10,448 times). As shown in Supplementary Figure 2 , the cooperative relationships among the productive authors are close, except for the group marked in yellow. There are several co-authorship groups, such as the red group with Saw SM as the core, the green group with Smith EL as the core, and the blue group with Mutti DO as the core.
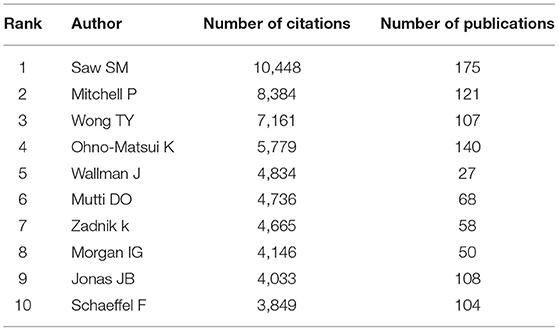
Table 2 . Top 10 most influential authors for myopia studies.
Distribution of Journals
Based on the retrieved results, the articles on myopia research were distributed among 164 journals. The top 10 journals that published articles on this topic are listed in Table 3 . According to the citations, Investigative Ophthalmology & Visual Science and Ophthalmology ranked first and second, respectively. The Journal of Cataract and Refractive Surgery published the largest number of myopia articles (834 papers), followed by Investigative Ophthalmology & Visual Science . Among the top 10 journals, eight were from the USA, one was from the United Kingdom, and one from Germany.
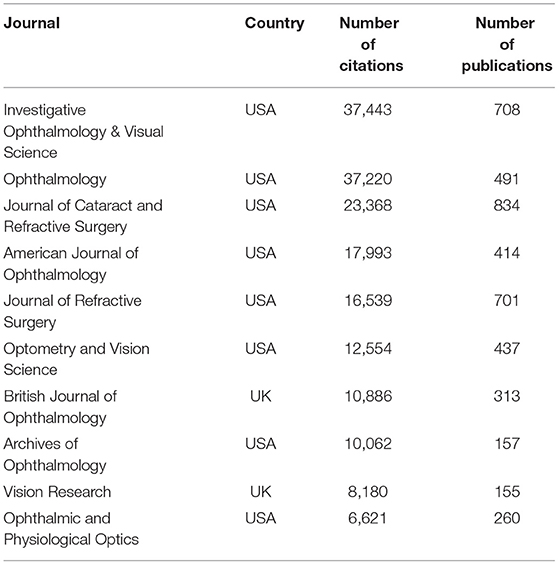
Table 3 . Top 10 influential source journals for myopia studies.
Distribution of Organizations
As shown in Table 4 , the top 10 organizations published 2,161 articles. Citation analysis showed that the National University of Singapore had 14,968 citations and ranked first. According to the publications, National University of Singapore and Sun Yat-sen University ranked first with 285 publications. The University of Melbourne, with 264 articles, ranked third. In the knowledge domain map of collaboration among main research organizations, 45 countries, 6 clusters, and 874 links were displayed and selected. As shown in Supplementary Figure 3 , the National University of Singapore has the highest number (35 links) and the strongest link strength (629).
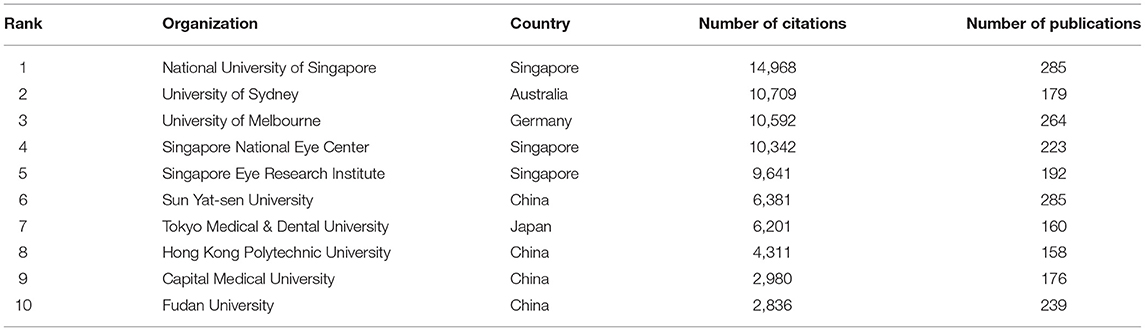
Table 4 . Top 10 influential organizations for myopia studies.
Top Cited Publications
The top 10 cited references are summarized in Table 5 . The top 10 papers were co-cited over 6,000 times in total, and the first was co-cited more than 800 times, while the 10th was cited 516 times. Additionally, the fifth paper was the only one published before the year 2000 cited 538 times. The top 10 cited references mainly focused on the prevalence and risk factors of myopia, which is consistent with the latest burst keyword.
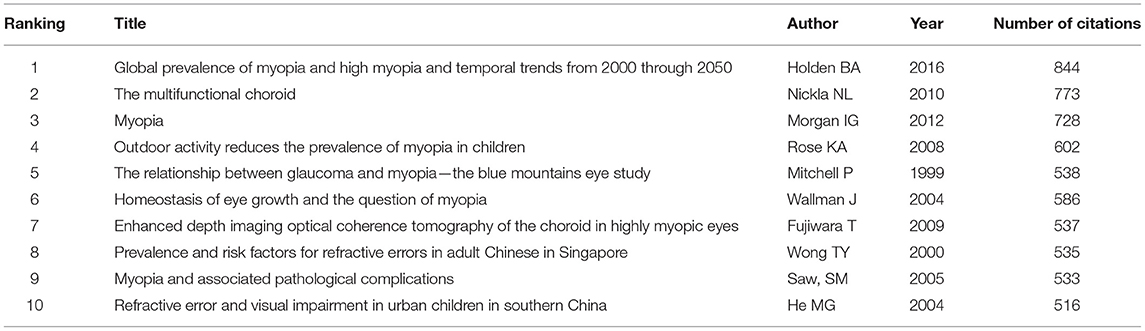
Table 5 . Top 10 cited papers in myopia citation network.
Myopia Research Keywords and Tendency
Through co-occurrence analysis, the keywords were visualized by density network map ( Figure 2 ). The keyword “ in-situ keratomileusis,” “prevalence,” and “photorefractive keratectomy” turned out to be significant. These keywords were the core keywords in myopia research. The top 29 keywords with the strongest citation bursts were extracted via keyword burst analysis from 1990 to 2020 ( Figure 3 ). “Chick,” the first keyword detected, appeared in 1990 and lasted for 12 years. Among the 29 keywords, “photorefractive keratectomy” had the highest burst strength (114.58) in the steady development stage. The latest keywords in the rapid development stage were “myopia control” and “trend.”
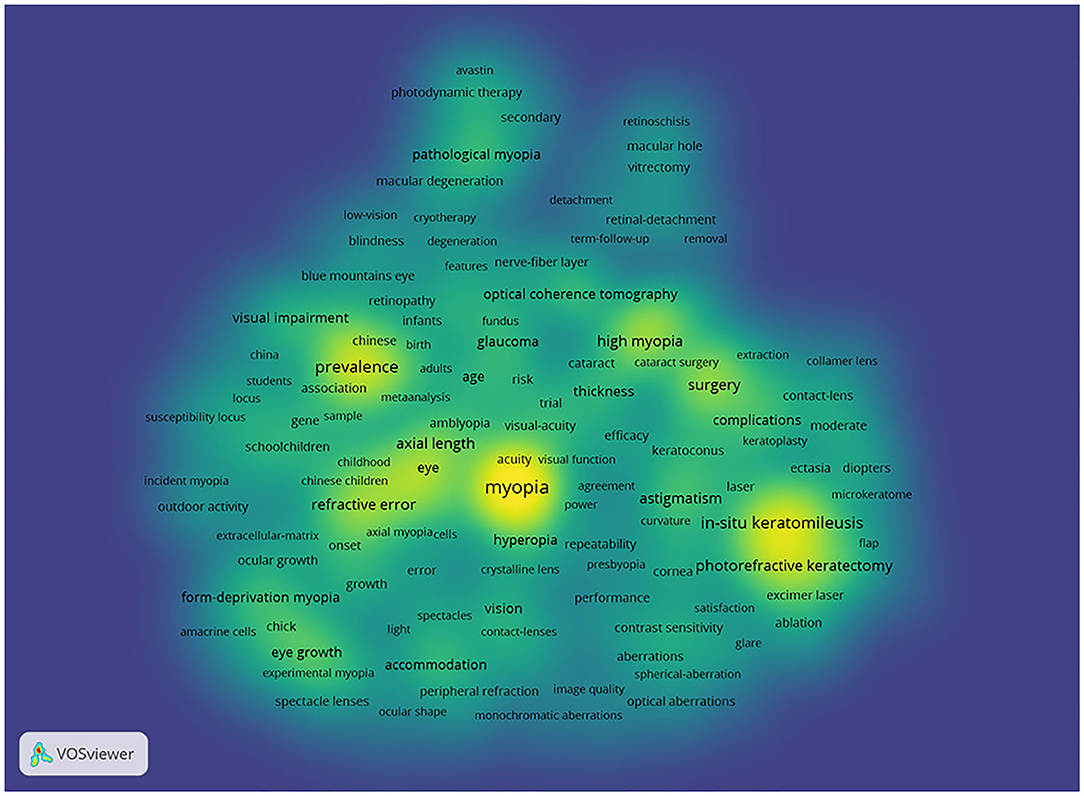
Figure 2 . Density visualization for keywords in co-occurrence network map. Each keyword in the density visualization has colors that indicates its appearing frequency. Keywords in yellow emerge more frequently, while green emerge blue less frequently.
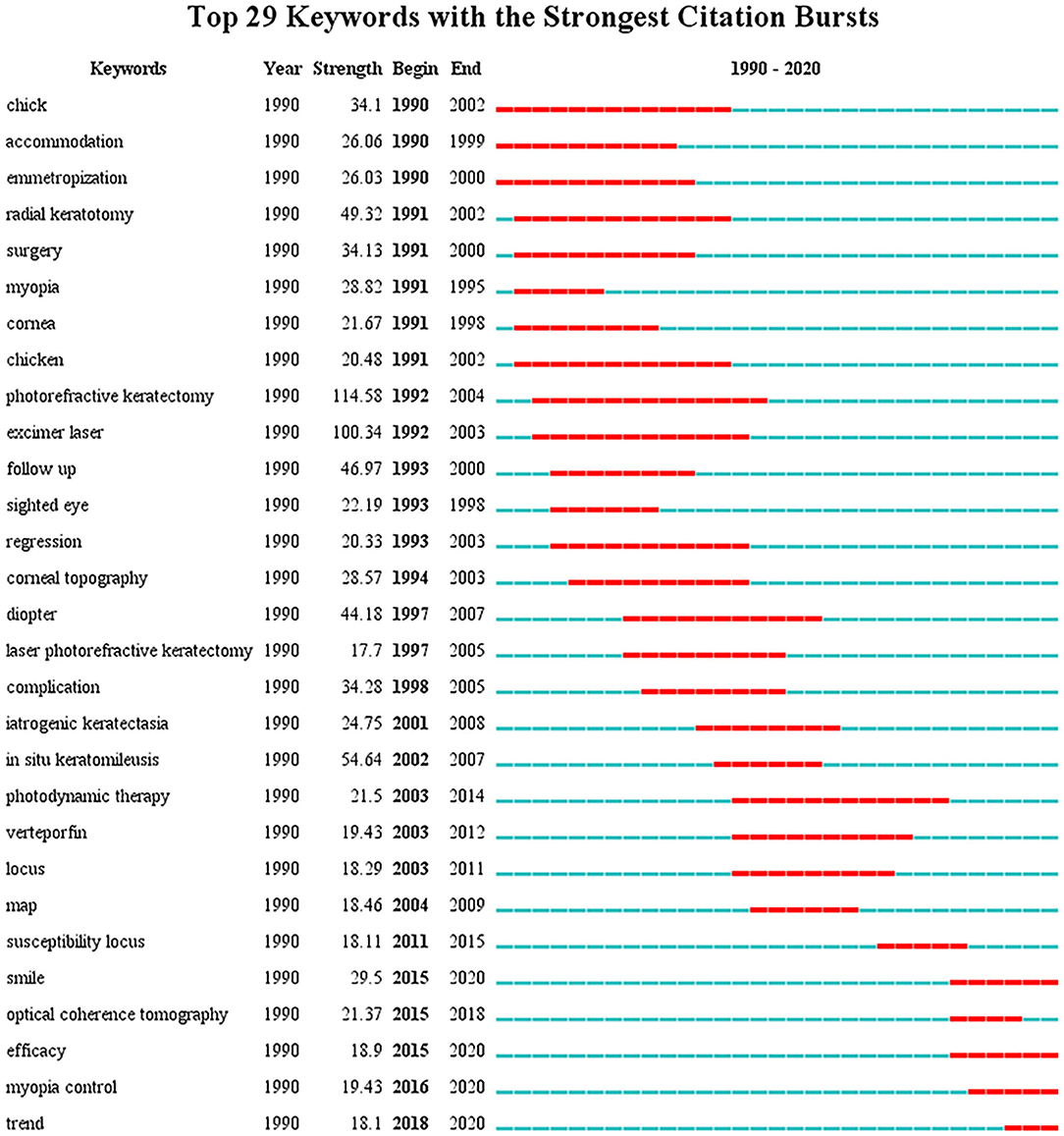
Figure 3 . The top 29 keywords with the strongest citation bursts in myopia research from 1990 to 2020. The blue lines represent the base timeline, while the red segments represent the burst duration of the keywords.
Myopia Research Citation Network
Figure 4 shows the main publication citation network of myopia. Based on the clustering function, each publication would be assigned to six research focuses. Each color marks a group. Each direction has its own citation network, which consists of publications that are strongly linked to each other.
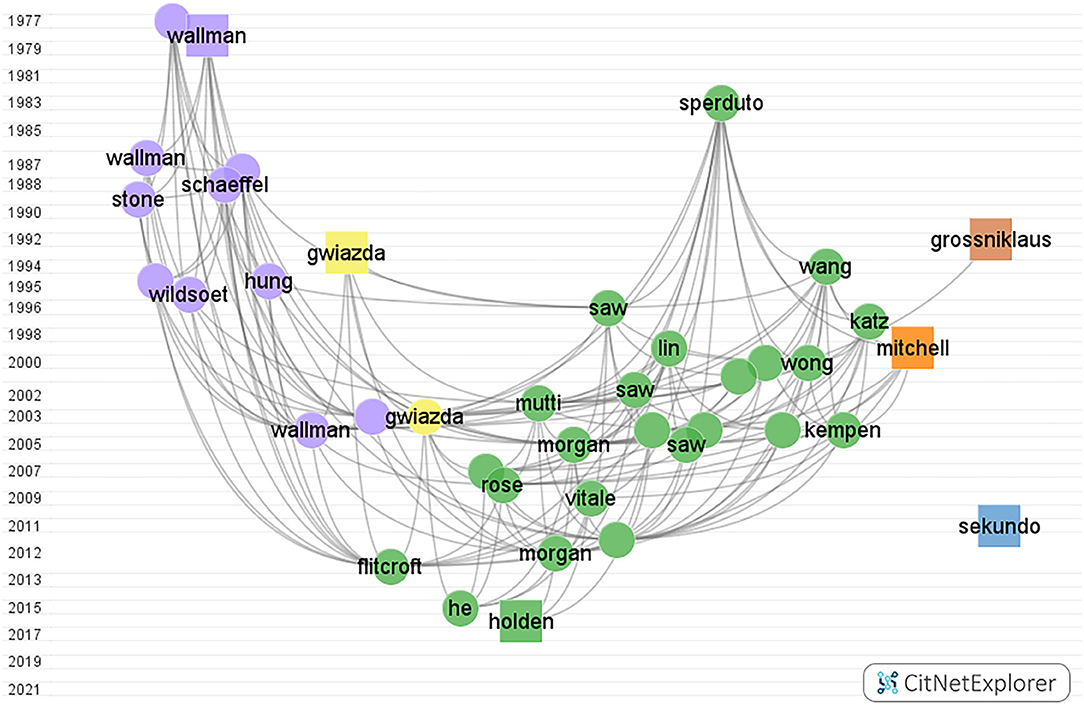
Figure 4 . Myopia citation network graph from CitNetExplorer from 1900 to 2000. The vertical axis coordinates indicate the publication year. Each dot/square indicates a publication which is labeled with the last name of the first author. Each color marks a group. Group 1 to group 6, in turn, were colored green, blue, purple, yellow, orange, and brown, respectively. The square represents the publication with the highest citation score in each group.
The color green represents the prevalence and risk factors of myopia group, containing 2,711 publications, and almost 32% of the total citation score. The color blue represents the surgical control of myopia group with 3,059 publications, and the total citation score was 34,557. The color purple represents the pathogenesis of myopia group, where 1,456 articles were found within the network. The color yellow represents the optical interventions of myopia group. The color orange represents the myopia and glaucoma group. The color brown represents the pathological myopia group.
Supplementary Figures 4 – 9 show the citation network of each of the six research focuses.
Bibliometric analysis is one of the most prominent methods for researchers to identify and predict new trends in potential topics. Moreover, it has been widely recognized as an alternative tool for evaluating academically detailed information in the library and information science. There has been some studies on myopia, but their coverages were limited in a single area of myopia research and did not include keywords bursts in its analysis ( 15 , 16 ). In this study, we conducted a comprehensive bibliometric analysis of the literature available on myopia from 1900 to 2020; six groups were identified within the citation network, and keywords bursts detection was performed.
Global Contribution in Myopia Research
Trend variations in publication quantity can reflect changes in knowledge on a certain subject. The number of documents on myopia studies has been through three stages: the initial stage (before 1991), steady development stage (from 1991 to 2011), and rapid development stage (after 2011). In the initial stage, the total publications were about 700, the annual average amount was about 7 papers. The increase in the global pattern of published papers was particularly prominent after the 1990's, which may be associated with a shift in focus toward newly developed techniques for refractive surgery with better safety and effectiveness ( 17 , 18 ).
International cooperation has become one of the main scientific research patterns among countries. In the current study, the United States was found to be the leading country in myopia research, accounting for 19.82% of total publications and the highest number of citations. According to the connection between various nodes, the United States attaches great importance to exchanges and cooperation in the academic community. This also explains why the United States has greater output to some extent. It can be speculated that adequate funding, advanced techniques, and equipment are essential factors. However, it is equally important that numerous authors from the United States produced high-quality research with good communication and collaboration with others. Smith EL of the University of Houston conducted animal experiments on myopia and explored the role of visual signals on refractive development. He stated that optical defocus can regulate eye growth and myopia progression by a small but statistically significant amount ( 19 ). In the initial stage, Curtin BJ was the most cited author, who was from the USA as well. The most cited article found that high myopia was associated with abnormal proteoglycans in sclera which changed the size and organization of collagen fibrils ( 20 ). In terms of the authors' analysis, Saw SM from Singapore was the most cited author in the steady development stage and the rapid development stage. In 2009, the article titled “Outdoor activity and myopia in Singapore teenage children” was published in the British Journal of Ophthalmology. This study suggested that outdoor activity may protect against the progression of myopia in children ( 21 ). Top source journals also came from the United States, with the Journal of Cataract and Refractive Surgery (JCRS) being the most prolific in publishing myopia research. Synthetically, Investigative Ophthalmology & Visual Science was the most influential journals, which ranked first of citations. As for the research institutions, among the top 10 institutions, eight institutions were located in Asia, which was in accordance with the increasing prevalence of myopia in East and Southeast Asia ( 22 , 23 ).
Focus in Myopia Research
Research focus represents the combination of clinical subjects and basic research and indicates the increasing or emerging themes in the field of myopia. In bibliometrics, the cluster function showed that all publications can be separated into six groups, and each group was summarized to a specific theme. With reference to the characteristics and status of myopia research, the following six groups are discussed.
Prevalence and Risk Factors of Myopia
The sharp rise in the myopic population increased its socioeconomic burden and posed a public health problem worldwide. Thus, the prevalence of myopia and its risk factors have gained widespread attention ( Supplementary Figure 1 , Green). Carrying out scientific epidemiological research on myopia is a mainstay for exploring related influencing factors for myopia, which are critical for intervening on its onset and progression. Before 1980, little was known about the distribution of myopia in the worldwide population. East and Southeast Asia showed the highest prevalence, reaching 80–90% at 18 years of age, which was much higher than that of Central Europe and Central Asia ( 24 , 25 ). A meta-analysis has suggested that by the year 2050, nearly 50% of the world's population will have myopia, and approximately 10% will be high myopic. This is the most cited paper in this myopia area published by Holden et al. in 2016 ( 2 ). The first prospective study of the risk factors for myopia, showed that earlier age of onset of myopia was a risk factor for the development of high myopia, which induced non-correctable visual impairment or blindness ( 3 ). The genetic pool has changed little over the past few decades, but the changes in the environmental factors may be responsible for the rapid increase in the prevalence of myopia ( 26 ). It seems that school myopia is multifactorial, strongly associated with intensive educational pressure and limited outdoor activities ( 27 ). In terms of educational level, there was a high prevalence of myopia in boys attending Orthodox schools in Israel compared with their peers attending secular schools ( 27 ). The mechanism involved is unclear; however, near-work requires more accommodation which may stimulate eye growth. According to the prevailing view, longer time spent outdoors can prevent myopia ( 28 ). The available data revealed that high-intensity outdoor light may act as a protective factor by adding retinal dopamine concentrations and thus preventing myopia ( 29 ).
Surgical Control of Myopia
With the development of laser technology in modern ophthalmic surgery, refractive surgery is an important research area to improve the refractive status ( 30 ) ( Supplementary Figure 5 , Blue). Refractive surgery, a safe and effective measure that corrects refractive errors, is generally not recommended until refractive development has stabilized around the age of eighteen. The first case of radial keratotomy surgery was reported by Fyodorov in 1979; however, it has been replaced by cornea laser surgery owing to its lower security and poor efficiency ( 31 ). The keratorefractive surgery revolution began with surface ablation techniques. Surface ablation is potentially suitable for high myopia and thin corneas due to the relatively thicker residual stromal thickness ( 32 ). Nonetheless, corneal haze and myopic regression may be more common after surface ablation ( 33 ). Laser-assisted in situ keratomileusis (LASIK) is a new revolution, which has been the standard refractive surgery used for treating myopia since the 1990's ( 34 ). A review of LASIK outcomes was published in 2016 by Sandoval et al. ( 35 ). The authors reported that the spherical equivalent refraction (SE) and the uncorrected distance visual acuity of eyes both obtained pretty good correction effects and up to 98.8% of patients were satisfied with their outcome. However, complications associated with this procedure are not rare, such as free cap, buttonhole flap ( 36 , 37 ). Presently, small-incision lenticule extraction (SMILE) has emerged as a novel surgery for myopia with the introduction of the femtosecond laser platform. The most frequently cited article in this cluster was published in 2011 and written by Sekundo et al. ( 38 ). The authors acknowledged that SMILE was a promising new minimally invasive procedure. SMILE has shown a reduced degree of dry eye symptoms and higher-order aberrations relative to LASIK ( 39 , 40 ). These advantages may stem from small side cut and lower laser energy which retains corneal nerve and reduce inflammatory responses to a large extent ( 41 , 42 ). Refractive surgery has already achieved excellent visual outcomes; however, the next challenge for clinicians is to choose the best refractive surgery method for each patient. Recently, with the addition of artificial intelligence in preoperative evaluation, data derived from corneal topography, biometry, and aberrometry can optimize customized refractive surgical strategies ( 43 , 44 ). Based on the keywords, myopia correction remains centered on the corneal refractive operation. Intraocular surgery, which avoids the risk of corneal ectasia, is also developing rapidly ( 45 ).
Pathogenesis of Myopia
At present, one of the areas that require further studies is the pathogenesis of myopia ( Supplementary Figure 6 , Violet). The mechanisms underlying axial elongation may provide scientific clues for the prevention and control of this global epidemic. From birth to adolescence, the eye achieves a close match between the power of its optics and its axial length, with images that are focused on the retina without accommodative effort (emmetropia) ( 46 ). Interestingly, Rucker FJ discovered that the retina may be using contrast perception to decode the sign of defocus using contrast perception. The chromatic signals of longitudinal chromatic aberration may rely on cone modulation to provide a direction signal for accommodation and eye growth ( 47 , 48 ). Within the past several decades, it has become clear that alterations in the visual experience can provoke myopia in animal models ( 19 , 49 ). For instance, form deprivation causes axial myopia through reduced visual stimulation, which is different from defocused-myopia related to the central system ( 50 ). The most frequently cited publication is the paper written by Wallman et al.. The authors reported that reading for long periods may disturb the homeostasis in the posterior globe, resulting in scleral remolding ( 51 ). The advances in clinical and basic experiments are mainly in the posterior ocular segment ( 52 – 54 ). Recently, Zhou et al. proposed a hypothesis that scleral hypoxia is a target for myopia control in 2018 ( 54 ). They speculated that special visual stimulation regulates the choroidal blood, thus initiating scleral hypoxia, leading to the onset and progression of myopia and axial elongation. Therefore, choroidal blood perfusion might be a “rapid predictive index” for myopia management ( 55 ). However, despite the progress, the chain of events of choroidal signals and scleral targets are still largely unknown. These clues may direct researchers to improve the understanding of myopia through the expansion of omics and big data analysis. In addition, some studies showed that atropine was found to increase the release of dopamine, strengthen the sclera, and increase the choroidal blood ( 56 , 57 ).
Optical Interventions of Myopia
As for group 4, the frequently cited articles were related to the optical interventions study ( Supplementary Figure 7 , Yellow). This group was led by the cross-sectional study by Millodot et al., published in 1981, in which the effect was measured between peripheral refraction and ametropia, where peripheral hyperopic defocus accelerated the onset of myopia ( 58 ). The first optical intervention was based on the reasoning that there was a relationship between myopia and excessive accommodation. The use of single-vision lenses (SVLs) was the mainstream method to correct myopia; however, SVLs poorly controlled myopia progression. It is not possible to clear the causality between peripheral retinal defocus and central myopia. However, it has been widely recognized that peripheral hyperopia can promote myopia progression ( 19 , 59 ). Thus, peripheral defocus spectacle lenses (PDSL) and orthokeratology that were precisely designed to reduce peripheral retinal defocus, were effective ( 60 , 61 ). The most cited publication was the article by Jane Gwiazda et al., which was published in 2003 in Investigative Ophthalmology & Visual Science ( 62 ). The authors reported that compared with SVLs, PALs limited the progression of myopia during the 1st year. The evaluation of visual quality in the human eye has always been an important issue in the field of ophthalmology and visual optics, which generally focus on the correlation analysis of visual acuity, wavefront aberrations, and contrast sensitivity ( 63 – 65 ). Previous research has indicated that visual quality was negatively related to the degree of myopia. It decreases gradually and concomitantly as the degree of myopia increases, while contrast sensitivity decreases and higher-order aberrations increase ( 66 , 67 ).
Myopia and Glaucoma
The connection between myopia and glaucoma clinically has also been a significant research topic in recent years ( Supplementary Figure 8 , Orange). Glaucoma is the leading cause of irreversible blindness worldwide, and primary open-angle glaucoma (POAG) is the major type of glaucoma. Glaucoma is strongly linked with the onset and progression of myopia, with homogeneity in structural and functional changes ( 68 ). The most cited paper was published in 1999 in Ophthalmology by Mitchell et al. and was ranked fifth in the top 10 cited publications ( 69 ). The study revealed that myopia, an independent risk, increased the prevalence of glaucoma by 2 or 3-fold. A study from Korea found a positive trend between increased myopic refractive error and POAG prevalence ( 70 ). Recently, a meta-analysis by Ahnul Ha et al. corroborated this finding: every diopter in myopia increases the risk of glaucoma by ~20% ( 71 ). Thus, high myopia is now regarded as a risk factor for glaucoma ( 72 – 74 ). With increased axial length, high myopia appears to have optic disc morphological changes and optic nerve fiber layer defects, accelerating visual field defects ( 72 , 73 ). In this group, the newest, most cited citation centers on myopia-related optic disc changes. Saw SM et al. reported that tilted discs and peripapillary atrophy were common in Singaporean adolescent children, which were similar to the pathological changes in glaucoma ( 75 ). Retinal degeneration makes it difficult to detect glaucoma with severe myopia, which requires a myopic normative database for analysis ( 76 ). Taken together, there is a need for a multimodal approach combining structural images with functional assessments to overcome the clinical diagnostic dilemmas of myopic eyes with glaucoma ( 77 ).
Pathological Changes of Myopia
Pathological myopia has gained attention because of its sustained axial elongation and irreversible fundus degeneration that leads to severe vision loss ( Supplementary Figure 9 , Brown). This cluster focuses on myopic maculopathy, especially in myopic choroidal neovascularization (mCNV) ( 78 ). The reasons behind the development of myopic maculopathy are not clear, but evidence has shown that excessive axial elongation weakens the retina, choroid, and sclera, which is accompanied by vascular complications and degeneration ( 79 , 80 ). Curtin and associates first proposed five fundus changes in myopia associated with axial elongation in 1970 ( 81 ). This classification did not cover all myopic maculopathy lesions. The development of fundus imaging technology facilitated a clearer visualization of myopic maculopathy. Grossniklaus et al. published an article in 1992 that described the pathological changes in pathological myopia and was the most cited paper in this group ( 82 ). A simplified classification system was proposed by Ohno-Matsui et al. ( 83 ). In this system, myopic maculopathy lesions were classified into five categories: no myopic retinal lesions (category 0), tessellated fundus only (category 1), diffuse chorioretinal atrophy (category 2), patchy chorioretinal atrophy (category 3), and macular atrophy (category 4), in combination with the three “plus signs” of lacquer crack, myopic choroidal neovascularization, and the Fuchs spot. Choroidal neovascularization may develop in 5–10% of individuals with pathological myopia ( 84 ), which is easily diagnosed using optical coherence tomography, optical coherence tomography angiography, and fundus fluorescein angiography. Photodynamic therapy with verteporfin (vPDT) was the first approved treatment for mCNV. Nevertheless, vPDT also resulted in chorioretinal atrophy and influenced the final visual outcome ( 85 , 86 ). In recent years, intravitreal anti-endothelial growth factor (anti-VEGF) injection has become the first-line treatment for CNV secondary to pathological myopia ( 87 ). Anti-VEGF agents, such as ranibizumab and aflibercept, can control neovascularization and reduce macular edema, thereby improving visual acuity ( 88 ). However, patients do not acquire significant benefits in long-term vision with this treatment due to the development of mCNV-related macular atrophy ( 89 ).
Tendency in Myopia Research
The strongest citation burst keywords were considered the indicators of research trends in basic and clinical research. As a result of the fewer numbers of annual average publications, no distinct research trend was observed in the initial stage. Despite this, the most cited paper showed that lid fusion led to elongation of the eye globe and varying degrees of myopia in monkeys published in 1977 ( 90 ).
In the steady development stage and rapid development stage, we conducted the keywords burst to explore myopia tendency and frontiers. According to the keyword co-occurrence chronology, the most prominent keywords in steady development stage are “photorefractive keratectomy,” “excimer laser,” and “ in situ keratomileusis,” indicating that the study focus was refractive surgery. Experts such as Da Vinci proposed the first theories as to the source of refractive errors long ago. At the Aerospace Medical Association, an intervention reported excimer laser can be used to change the corneal shape, piqued experts' curiosity ( 91 ). McDonald and colleagues became the first to utilize an excimer laser in the human eye with myopia in 1988 ( 92 ). As the number of surgeries rose, the drawbacks of photorefractive keratectomy, started to emerge such as postoperative pain and corneal haze. In the 1990's, Pallikaris with colleagues proposed a novel surgical procedure (LASIK) that merged the microkeratome with the excimer laser, and it has now become a wildly used refractive technique ( 93 ). In addition to clinical studies, animal studies had a high profile within the steady development stage. Notably, the keyword chick appeared twice in the analysis. Several animal studies have demonstrated environmental factors can exert a significant effect on myopia. In this regard, we found that Wildsoet CF is the most cited author at the second period. Most attention was drawn to an article published in 1994 showing that hyperopic defocus induced with negative lens led to increased ocular length and choroidal chinning, whereas myopic defocus induced with positive lens led to decreased ocular elongation and choroidal thickening ( 94 ). According to recent studies by Wildsoet CF, the Bone Morphogenetic Proteins (BMP 2, 4, and 7) gene is down-regulated with form-deprivation and hyperopic defocus, and up-regulated with myopic defocus in chicks, which exhibited regional differences in retinal pigment epithelium. Consequently, it is tempting to speculate that BMPs played a crucial role in ocular growth signaling ( 95 , 96 ).
During the rapid development stage, we have observed that a new chapter in refractive surgery was opened with the application of the femtosecond laser in ophthalmology. SMILE has been the most recent, strongest burst, which has been approved by the Food and Drug Administration for the treatment of myopia and astigmatism preventing iatrogenic dry eye and allowed better spherical aberration control ( 97 , 98 ). Beyond 2020, refractive surgery might be guided by artificial intelligence to make precise decisions regarding surgery details and improve the quality of the retinal image ( 99 , 100 ). In the third phase, the keywords “myopia control” and “trend” show that there is an urgent need for society to take interventions on myopia, which are the new research hotspots in this field. With the rapidly growing prevalence of myopia already at epidemic levels in some regions and imposing a heavy public health burden ( 5 ), the scientific interest in myopia control is growing with each passing day. The amount of scientific papers has increased excessively concerning myopia control. Myopia management strategies consist of two parts: the prevention of myopia onset and slowing the progression of myopia ( 99 ). The current control measures, including optical, pharmacological, behavioral, and surgical interventions. On the basis of a series of influential studies, increased time outdoors could preclude high engagement in near-work activities and exposure to ultraviolet radiation, which is more meaningful in preventing myopia ( 21 ). A recent review suggested that the changes in SE and axial length were better in the outdoor group than that in the control group ( 101 ). Studies using animal models reported that bright light may play an inhibitory role in response to imposed form-deprivation ( 102 ). When compared with other measures, wearing optical devices is a convenient method that reduces the peripheral hyperopic defocus to limit myopic progression. Substantial evidence from animal research indicates that hyperopic defocus induces axial elongation whereas myopic defocus inhibits the growth of axial elongation ( 103 ). For the present research outcomes, pharmacological treatment was insufficient to cluster into groups in the myopia field. Atropine is the most extensively studied medicine in slowing progression. Low-dose atropine (0.01%) seemed to be the most effective treatment and had a lower risk of rebound according to the Atropine for Treatment of Myopia (ATOM2) study ( 104 ). It was suggested that atropine may exert its function by altering choroidal thickness to reduce scleral proteoglycan synthesis ( 105 , 106 ). As for surgical interventions for the control of myopia, scleral reinforcement to slow ocular elongation has a long history. The revitalized interest arose from collagen cross-linking scleral strengthening (CCL) controlling scleral biochemistry which has involved animals only ( 107 ). Recently, Bullimore et al. reported that the potential benefits of myopia control outweigh the risks ( 108 ). Moreover, applying artificial intelligence to ocular data may provide a better approach for reducing public burden focusing on myopia control. Generally, the preventive strategies aim to avoid younger age of myopia onset or lower the risk of high myopia; therefore, the sooner the intervention, the better is the outcome and the impact on public health. In short, the efforts of myopia controls could have a profound impact on public health.
Strengths and Limitations
The present study is the first bibliometric analysis of myopia performed using the literature from the entire 20th century. To acquire deep insight into myopia research, VOSviewer was used to identify the hotspots and major clusters in this field. However, despite these advantages, several limitations should be noted in our study. The data were only retrieved from the WOS database and did not include other medical databases such as PubMed and Scopus. As reported, the WOS database has more accuracy in document type assignment than Scopus ( 109 ). The WOS was preferred over PubMed due to a unique citation report function ( 11 ). Regardless, the WOS database is the most commonly used reference database for bibliometric analysis.
Conclusions
Based on the bibliometric analysis, myopia has been growing as a core research area. United States has the most significant academic impact on myopia studies. The most productive and cited institution was the National University of Singapore. Saw SM is one of the key researchers in this field. The priority themes involved the prevalence and risk factors of myopia and surgical control of myopia. With the increasing prevalence of myopia, the interventions of myopia control are the potential research hotspot and pressing issue. Taken together, these analysis results should help researchers realize the current state and provide promising directions for future research.
Data Availability Statement
The datasets presented in this study can be found in online repositories. The names of the repository/repositories and accession number(s) can be found in the article/ Supplementary Material .
Author Contributions
MS and YD did this bibliometrics analysis and drafted manuscript. MS and JC organized the manuscript writing. QS searched strategy. YW reviewed the manuscript. All authors contributed to the article and approved the submitted version.
This research was funded by the National Natural Science Foundation of China, Grant No: 81873684.
Conflict of Interest
The authors declare that the research was conducted in the absence of any commercial or financial relationships that could be construed as a potential conflict of interest.
Publisher's Note
All claims expressed in this article are solely those of the authors and do not necessarily represent those of their affiliated organizations, or those of the publisher, the editors and the reviewers. Any product that may be evaluated in this article, or claim that may be made by its manufacturer, is not guaranteed or endorsed by the publisher.
Acknowledgments
The authors sincerely treasured the comments and suggestions from reviewers.
Supplementary Material
The Supplementary Material for this article can be found online at: https://www.frontiersin.org/articles/10.3389/fpubh.2022.846601/full#supplementary-material
1. Baird PN, Saw SM, Lanca C, Guggenheim JA, Smith EL, Zhou X, et al. Myopia. Nat Rev Dis Primers. (2020) 6:99. doi: 10.1038/s41572-020-00231-4
PubMed Abstract | CrossRef Full Text | Google Scholar
2. Holden BA, Fricke TR, Wilson DA, Jong M, Naidoo KS, Sankaridurg P, et al. Global prevalence of myopia and high myopia and temporal trends from 2000 through 2050. Ophthalmology. (2016) 123:1036–42. doi: 10.1016/j.ophtha.2016.01.006
3. Li J, Lanca C, Htoon HM, Wong YL, Nyunt SZ, Tan D, et al. High myopes in Singapore: 19-year progression from childhood to adulthood. Ophthalmology. (2020) 127:1768–70. doi: 10.1016/j.ophtha.2020.05.031
4. Saw SM, Gazzard G, Shih-Yen EC, Chua WH. Myopia and associated pathological complications. Ophthalmic Physiol Opt. (2005) 25:381–91. doi: 10.1111/j.1475-1313.2005.00298.x
5. Modjtahedi BS, Ferris FL 3rd, Hunter DG, Fong DS. Public Health Burden and Potential Interventions for Myopia. Ophthalmology. (2018) 125:628–30. doi: 10.1016/j.ophtha.2018.01.033
6. Edward WH. Statistical bibliography in relation to the growth of modern civilization. Economica . (1923) 112:585–6.
Google Scholar
7. Agarwal A, Durairajanayagam D, Tatagari S, Esteves SC, Harlev A, Henkel R, et al. Bibliometrics: tracking research impact by selecting the appropriate metrics. Asian J Androl. (2016) 18:296–309. doi: 10.4103/1008-682X.171582
8. Fagundes MVC, Teles EO, Vieira de Melo SAB, Freires FGM. Decision-making models and support systems for supply chain risk: literature mapping and future research agenda. Eur Res Manage Business Econ. (2020) 26:63–70. doi: 10.1016/j.iedeen.2020.02.001
CrossRef Full Text | Google Scholar
9. Peng X, Luo Z. A review of q-rung orthopair fuzzy information: bibliometrics and future directions. Artifi Intellig Rev. (2021) 54:3361–430. doi: 10.1007/s10462-020-09926-2
10. Lu K, Liao H, Kazimieras Zavadskas E. An overview of fuzzy techniques in supply chain management: bibliometrics, methodologies, applications and future directions. Technol Econ Dev Economy. (2021) 27:402–58. doi: 10.3846/tede.2021.14433
11. Falagas ME, Pitsouni EI, Malietzis GA, Pappas G. Comparison of PubMed, Scopus, Web of Science, and Google Scholar: strengths and weaknesses. FASEB J. (2008) 22:338–42. doi: 10.1096/fj.07-9492LSF
12. Van Eck NJ, Waltman L. CitNetExplorer: A new software tool for analyzing and visualizing citation networks. J Inform. (2014) 8:802–23. doi: 10.1016/j.joi.2014.07.006
13. Van Eck NJ, Waltman L. Citation-based clustering of publications using CitNetExplorer and VOSviewer. Scientometrics. (2017) 111:1053–70. doi: 10.1007/s11192-017-2300-7
14. Hu S, Alimire A, Lai Y, Hu H, Chen Z, Li Y. Trends and frontiers of research on cancer gene therapy from 2016 to 2020: a bibliometric analysis. Front Med (Lausanne). (2021) 8:740710. doi: 10.3389/fmed.2021.740710
15. Efron N, Morgan PB, Jones LW, Nichols JJ. Topical review: bibliometric analysis of the emerging field of myopia management. Optom Vis Sci. (2021) 98:1039–44. doi: 10.1097/OPX.0000000000001766
16. Alvarez-Peregrina C, Martinez-Perez C, Villa-Collar C, Sanchez-Tena MA. A bibliometric and citation network analysis of myopia genetics. Genes (Basel). (2021) 12:447. doi: 10.3390/genes12030447
17. Pérez-Santonja JJ, Bellot J, Claramonte P, Ismail MM, Alio JL. Laser in situ keratomileusis to correct high myopia. J Cataract Refractive Surg. (1997) 23:372–85. doi: 10.1016/S0886-3350(97)80182-4
18. Pallikaris IG, Siganos DS. Excimer laser in situ keratomileusis and photorefractive keratectomy for correction of high myopia. J Refract Corneal Surg. (1994) 10:498–510. doi: 10.3928/1081-597X-19940901-07
19. Smith EL 3rd, Kee CS, Ramamirtham R, Qiao-Grider Y, Hung LF. Peripheral vision can influence eye growth and refractive development in infant monkeys. Invest Ophthalmol Vis Sci . (2005) 46:3965–72. doi: 10.1167/iovs.05-0445
20. Curtin BJ, Iwamoto T, Renaldo DP. Normal and staphylomatous sclera of high myopia. An electron microscopic study. Arch Ophthalmol. (1979) 97:912–5. doi: 10.1001/archopht.1979.01020010470017
21. Dirani M, Tong L, Gazzard G, Zhang X, Chia A, Young TL, et al. Outdoor activity and myopia in Singapore teenage children. Br J Ophthalmol. (2009) 93:997–1000. doi: 10.1136/bjo.2008.150979
22. Saw S-M, Carkeet A, Chia K-S, Stone RA, Tan DTH. Component dependent risk factors for ocular parameters in Singapore Chinese children. Ophthalmology. (2002) 109:2065–71. doi: 10.1016/S0161-6420(02)01220-4
23. Fan DS, Lam DS, Lam RF, Lau JT, Chong KS, Cheung EY, et al. Prevalence, incidence, and progression of myopia of school children in Hong Kong. Invest Ophthalmol Vis Sci. (2004) 45:1071–5. doi: 10.1167/iovs.03-1151
24. Foster PJ, Jiang Y. Epidemiology of myopia. Eye (Lond). (2014) 28:202–8. doi: 10.1038/eye.2013.280
25. Morgan IG, French AN, Ashby RS, Guo X, Ding X, He M, et al. The epidemics of myopia: Aetiology and prevention. Prog Retin Eye Res. (2018) 62:134–49. doi: 10.1016/j.preteyeres.2017.09.004
26. Morgan IG, Wu PC, Ostrin LA, Tideman JWL, Yam JC, Lan W, et al. IMI risk factors for myopia. Invest Ophthalmol Vis Sci. (2021) 62:3. doi: 10.1167/iovs.62.5.3
27. Zylbermann R, Landau D, Berson D. The influence of study habits on myopia in Jewish teenagers. J Pediatr Ophthalmol Strabismus. (1993) 30:319–22. doi: 10.3928/0191-3913-19930901-12
28. Jonas JB, Xu L, Wei WB, Wang YX, Jiang WJ, Bi HS, et al. Myopia in China: a population-based cross-sectional, histological, and experimental study. Lancet. (2016) 388:20. doi: 10.1016/S0140-6736(16)31947-X
29. Landis EG, Chrenek MA, Chakraborty R, Strickland R, Bergen M, Yang V, et al. Increased endogenous dopamine prevents myopia in mice. Exp Eye Res. (2020) 193:107956. doi: 10.1016/j.exer.2020.107956
30. Kim T-i, Alió del Barrio JL, Wilkins M, Cochener B, Ang M. Refractive surgery. Lancet. (2019) 393:2085–98. doi: 10.1016/S0140-6736(18)33209-4
31. Fyodorov SN, Durnev VV. Operation of dosaged dissection of corneal circular ligament in cases of myopia of mild degree. Ann Ophthalmol. (1979) 11:1885–90.
PubMed Abstract | Google Scholar
32. Sanchez P, Moutsouris K, Pandolfi A. Biomechanical and optical behavior of human corneas before and after photorefractive keratectomy. J Cataract Refract Surg. (2014) 40:905–17. doi: 10.1016/j.jcrs.2014.03.020
33. Na KS, Chung SH, Kim JK, Jang EJ, Lee NR, Joo CK. Comparison of LASIK and surface ablation by using propensity score analysis: a multicenter study in Korea. Invest Ophthalmol Vis Sci . (2012) 53:7116–21. doi: 10.1167/iovs.12-9826
34. Sandoval HP, de Castro LE, Vroman DT, Solomon KD. Refractive surgery survey 2004. J Cataract Refract Surg . (2005) 31:221–33. doi: 10.1016/j.jcrs.2004.08.047
35. Sandoval HP, Donnenfeld ED, Kohnen T, Lindstrom RL, Potvin R, Tremblay DM, et al. Modern laser in situ keratomileusis outcomes. J Cataract Refract Surg. (2016) 42:1224–34. doi: 10.1016/j.jcrs.2016.07.012
36. Jain V, Mhatre K, Shome D. Flap buttonhole in thin-flap laser in situ keratomileusis: case series and review. Cornea. (2010) 29:655–8. doi: 10.1097/ICO.0b013e3181c377d5
37. Geggel HS. Treatment of lost flaps and slipped flaps. Int Ophthalmol Clin. (2008) 48:65–71. doi: 10.1097/IIO.0b013e31815eb96d
38. Sekundo W, Kunert KS, Blum M. Small incision corneal refractive surgery using the small incision lenticule extraction (SMILE) procedure for the correction of myopia and myopic astigmatism: results of a 6 month prospective study. Br J Ophthalmol. (2011) 95:335–9. doi: 10.1136/bjo.2009.174284
39. Ganesh S, Gupta R. Comparison of visual and refractive outcomes following femtosecond laser- assisted lasik with smile in patients with myopia or myopic astigmatism. J Refract Surg . (2014) 30:590–6. doi: 10.3928/1081597X-20140814-02
40. Kobashi H, Kamiya K, Shimizu K. Dry eye after small incision lenticule extraction and femtosecond laser-assisted LASIK: meta-analysis. Cornea . (2017) 36:85–91. doi: 10.1097/ICO.0000000000000999
41. Aristeidou A, Taniguchi EV, Tsatsos M, Muller R, McAlinden C, Pineda R, et al. The evolution of corneal and refractive surgery with the femtosecond laser. Eye Vis. (2015) 2:12. doi: 10.1186/s40662-015-0022-6
42. Riau AK, Angunawela RI, Chaurasia SS, Lee WS, Tan DT, Mehta JS. Early corneal wound healing and inflammatory responses after refractive lenticule extraction (ReLEx). Invest Ophthalmol Vis Sci. (2011) 52:6213–21. doi: 10.1167/iovs.11-7439
43. Cui T, Wang Y, Ji S, Li Y, Hao W, Zou H, et al. Applying machine learning techniques in nomogram prediction and analysis for SMILE treatment. Am J Ophthalmol. (2020) 210:71–7. doi: 10.1016/j.ajo.2019.10.015
44. Redd TK, Campbell JP, Chiang MF. Artificial intelligence for refractive surgery screening: finding the balance between myopia and hype-ropia. JAMA Ophthalmol. (2020) 138:526–7. doi: 10.1001/jamaophthalmol.2020.0515
45. Guell JL, Morral M, Kook D, Kohnen T. Phakic intraocular lenses part 1: historical overview, current models, selection criteria, and surgical techniques. J Cataract Refract Surg. (2010) 36:1976–93. doi: 10.1016/j.jcrs.2010.08.014
46. Bradley DV, Fernandes A, Lynn M, Tigges M, Boothe RG. Emmetropization in the rhesus monkey (Macaca mulatta): birth to young adulthood. Invest Ophthalmol Vis Sci. (1999) 40:214–29.
47. Rucker FJ, Wallman J. Chick eyes compensate for chromatic simulations of hyperopic and myopic defocus: evidence that the eye uses longitudinal chromatic aberration to guide eye-growth. Vision Res. (2009) 49:1775–83. doi: 10.1016/j.visres.2009.04.014
48. Rucker FJ. The role of luminance and chromatic cues in emmetropisation. Ophthalmic Physiol Opt . (2013) 33:196–214. doi: 10.1111/opo.12050
49. Wallman J, Turkel J, Trachtman J. Extreme myopia produced by modest change in early visual experience. Science. (1978) 201:1249–51. doi: 10.1126/science.694514
50. Kee CS, Marzani D, Wallman J. Differences in time course and visual requirements of ocular responses to lenses and diffusers. Invest Ophthalmol Vis Sci. (2001) 42:575–83. Available online at: https://iovs.arvojournals.org/article.aspx?articleid=2123787
51. Wallman J, Winawer J. Homeostasis of eye growth and the question of myopia. Neuron. (2004) 43:447–68. doi: 10.1016/j.neuron.2004.08.008
52. McBrien N. Role of the sclera in the development and pathological complications of myopia. Prog Retinal Eye Res. (2003) 22:307–38. doi: 10.1016/S1350-9462(02)00063-0
53. Nickla DL, Wallman J. The multifunctional choroid. Prog Retin Eye Res. (2010) 29:144–68. doi: 10.1016/j.preteyeres.2009.12.002
54. Wu H, Chen W, Zhao F, Zhou Q, Reinach PS, Deng L, et al. Scleral hypoxia is a target for myopia control. Proc Natl Acad Sci USA. (2018) 115:E7091–100. doi: 10.1073/pnas.1721443115
55. Zhou X, Ye C, Wang X, Zhou W, Reinach P, Qu J. Choroidal blood perfusion as a potential “rapid predictive index” for myopia development and progression. Eye Vis (Lond). (2021) 8:1. doi: 10.1186/s40662-020-00224-0
56. Upadhyay A, Beuerman RW. Biological mechanisms of atropine control of myopia. Eye Contact Lens. (2020) 46:129–35. doi: 10.1097/ICL.0000000000000677
57. Cristaldi M, Olivieri M, Pezzino S, Spampinato G, Lupo G, Anfuso CD, et al. Atropine differentially modulates ECM production by ocular fibroblasts, and its ocular surface toxicity is blunted by colostrum. Biomedicines. (2020) 8:78. doi: 10.3390/biomedicines8040078
58. Millodot M. Effect of ametropia on peripheral refraction. Am J Optom Physiol Opt. (1981) 58:691–5. doi: 10.1097/00006324-198109000-00001
59. Smith EL, Hung LF, Huang J. Relative peripheral hyperopic defocus alters central refractive development in infant monkeys. Vision Res. (2009) 49:2386–92. doi: 10.1016/j.visres.2009.07.011
60. Sankaridurg P, Donovan L, Varnas S, Ho A, Chen X, Martinez A, et al. Spectacle lenses designed to reduce progression of myopia: 12-month results. Optom Vis Sci . (2010) 87:631–41. doi: 10.1097/OPX.0b013e3181ea19c7
61. Swarbrick HA, Alharbi A, Watt K, Lum E, Kang P. Myopia control during orthokeratology lens wear in children using a novel study design. Ophthalmology. (2015) 122:620–30. doi: 10.1016/j.ophtha.2014.09.028
62. Gwiazda J, Hyman L, Hussein M, Everett D, Norton TT, Kurtz D, et al. A randomized clinical trial of progressive addition lenses versus single vision lenses on the progression of myopia in children. Invest Ophthalmol Vis Sci. (2003) 44:1492–500. doi: 10.1167/iovs.02-0816
63. Shamir RR, Friedman YG, Joskowicz L, Mimouni M, Blumenthal EZ. The influence of varying the number of characters per row on the accuracy and reproducibility of the ETDRS visual acuity chart. Graefes Arch Clin Exp Ophthalmol. (2016) 254:971–6. doi: 10.1007/s00417-015-3252-3
64. LN T. Wavefront data reporting and terminology. J Refractive Surg . (2001) 17:S578–83. doi: 10.3928/1081-597X-20010901-14
65. Pang Y, Allen M, Robinson J, Frantz KA. Contrast sensitivity of amblyopic eyes in children with myopic anisometropia. Clin Exp Optom. (2019) 102:57–62. doi: 10.1111/cxo.12817
66. Stoimenova BD. The effect of myopia on contrast thresholds. Invest Ophthalmol Vis Sci. (2007) 48:2371–4. doi: 10.1167/iovs.05-1377
67. Liou SW, Chiu CJ. Myopia and contrast sensitivity function. Curr Eye Res. (2001) 22:81–4. doi: 10.1076/ceyr.22.2.81.5530
68. Kim MJ, Park KH, Kim CY, Jeoung JW, Kim SH. The distribution of intraocular pressure and associated systemic factors in a Korean population: the Korea National Health and Nutrition Examination Survey. Acta Ophthalmol. (2014) 92:e507–13. doi: 10.1111/aos.12327
69. Mitchell P, Hourihan F, Sandbach J, Jin Wang J. The relationship between glaucoma and myopia. Ophthalmology. (1999) 106:2010–5. doi: 10.1016/S0161-6420(99)90416-5
70. Shim SH, Sung KR, Kim JM, Kim HT, Jeong J, Kim CY, et al. The prevalence of open-angle glaucoma by age in myopia: The Korea national health and nutrition examination survey. Curr Eye Res. (2017) 42:65–71. doi: 10.3109/02713683.2016.1151053
71. Ha A, Kim CY, Shim SR, Chang IB, Kim YK. Degree of myopia and glaucoma risk: a dose-response meta-analysis. Am J Ophthalmol. (2021) 236:107–19. doi: 10.1016/j.ajo.2021.10.007
72. Park HY, Lee K, Park CK. Optic disc torsion direction predicts the location of glaucomatous damage in normal-tension glaucoma patients with myopia. Ophthalmology. (2012) 119:1844–51. doi: 10.1016/j.ophtha.2012.03.006
73. Samarawickrama C, Mitchell P, Tong L, Gazzard G, Lim L, Wong TY, et al. Myopia-related optic disc and retinal changes in adolescent children from singapore. Ophthalmology. (2011) 118:2050–7. doi: 10.1016/j.ophtha.2011.02.040
74. Biswas S, Jhanji V, Leung CK. Prevalence of glaucoma in myopic corneal refractive surgery candidates in Hong Kong China. J Refract Surg. (2016) 2016:32298–304. doi: 10.3928/1081597X-20160229-01
75. Chang L, Pan CW, Ohno-Matsui K, Lin X, Cheung GC, Gazzard G, et al. Myopia-related fundus changes in Singapore adults with high myopia. Am J Ophthalmol. (2013) 155:991–9.e1. doi: 10.1016/j.ajo.2013.01.016
76. Biswas S, Lin C, Leung CK. Evaluation of a myopic normative database for analysis of retinal nerve fiber layer thickness. JAMA Ophthalmol. (2016) 134:1032–9. doi: 10.1001/jamaophthalmol.2016.2343
77. Tan NYQ, Sng CCA, Jonas JB, Wong TY, Jansonius NM, Ang M. Glaucoma in myopia: diagnostic dilemmas. Br J Ophthalmol. (2019) 103:1347–55. doi: 10.1136/bjophthalmol-2018-313530
78. Wong YL, Sabanayagam C, Ding Y, Wong CW, Yeo AC, Cheung YB, et al. Prevalence, risk factors, and impact of myopic macular degeneration on visual impairment and functioning among adults in Singapore. Invest Ophthalmol Vis Sci. (2018) 59:4603–13. doi: 10.1167/iovs.18-24032
79. Ohno-Matsui K, Yoshida T, Futagami S, Yasuzumi K, Shimada N, Kojima A, et al. Patchy atrophy and lacquer cracks predispose to the development of choroidal neovascularisation in pathological myopia. Br J Ophthalmol. (2003) 87:570–3. doi: 10.1136/bjo.87.5.570
80. Yokoi T, Jonas JB, Shimada N, Nagaoka N, Moriyama M, Yoshida T, et al. Peripapillary diffuse chorioretinal atrophy in children as a sign of eventual pathologic myopia in adults. Ophthalmology. (2016) 123:1783–7. doi: 10.1016/j.ophtha.2016.04.029
81. Curtin BJ, Karlin DB. Axial length measurements and fundus changes of the myopic eye. Am J Ophthalmol. (1971) 71:42–53. doi: 10.1016/0002-9394(71)91092-0
82. Grossniklaus HE, Green WR. Pathologic findings in pathologic myopia. Retina. (1992) 12:127–33. doi: 10.1097/00006982-199212020-00009
83. Ohno-Matsui K, Kawasaki R, Jonas JB, Cheung CM, Saw SM, Verhoeven VJ, et al. International photographic classification and grading system for myopic maculopathy. Am J Ophthalmol. (2015) 159:877–83.e7. doi: 10.1016/j.ajo.2015.01.022
84. Wong TY, Ferreira A, Hughes R, Carter G, Mitchell P. Epidemiology and disease burden of pathologic myopia and myopic choroidal neovascularization: an evidence-based systematic review. Am J Ophthalmol. (2014) 157:9–25.e12. doi: 10.1016/j.ajo.2013.08.010
85. Hayashi K, Ohno-Matsui K, Teramukai S, Shimada N, Moriyama M, Hayashi W, et al. Comparison of visual outcome and regression pattern of myopic choroidal neovascularization after intravitreal bevacizumab or after photodynamic therapy. Am J Ophthalmol. (2009) 148:396–408. doi: 10.1016/j.ajo.2009.03.026
86. Parodi MB, Da Pozzo S, Ravalico G. Angiographic features after photodynamic therapy for choroidal neovascularisation in age related macular degeneration and pathological myopia. Br J Ophthalmol. (2003) 87:177–83. doi: 10.1136/bjo.87.2.177
87. Cohen SY. Anti-VEGF drugs as the 2009 first-line therapy for choroidal neovascularization in pathologic myopia. Retina. (2009) 29:1062–6. doi: 10.1097/IAE.0b013e3181b1bb1a
88. Ikuno Y, Ohno-Matsui K, Wong TY, Korobelnik JF, Vitti R, Li T, et al. Intravitreal aflibercept injection in patients with myopic choroidal neovascularization: the MYRROR study. Ophthalmology. (2015) 122:1220–7. doi: 10.1016/j.ophtha.2015.01.025
89. Hamilton RD, Clemens A, Minnella AM, Lai TYY, Dai H, Sakamoto T, et al. Real-world effectiveness and safety of ranibizumab for the treatment of myopic choroidal neovascularization: Results from the LUMINOUS study. PLoS ONE. (2020) 15:e0227557. doi: 10.1371/journal.pone.0227557
90. Wiesel TN, Raviola E. Myopia and eye enlargement after neonatal lid fusion in monkeys. Nature. (1977) 266:66–8. doi: 10.1038/266066a0
91. Taboada J, Archibald CJ. An extreme sensitivity in the corneal epithelium to far UV-ArF excimer laser pulses. In: Proceedings of the Aerospace Medical Association, 52 Annual Meeting . Washington, DC (1981).
92. McDonald MB, Kaufman HE, Frantz JM, Shofner S, Salmeron B, Klyce SD. Excimer laser ablation in a human eye. Case report. Arch Ophthalmol. (1989) 107:641–2. doi: 10.1001/archopht.1989.01070010659013
93. Pallikaris IG, Papatzanaki ME, Stathi EZ, Frenschock O, Georgiadis A. Laser in situ keratomileusis. Lasers Surg Med. (1990) 10:463–8. doi: 10.1002/lsm.1900100511
94. Wildsoet C, Wallman J. Choroidal and scleral mechanisms of compensation for spectacle lenses in chicks. Vision Res. (1995) 35:1175–94. doi: 10.1016/0042-6989(94)00233-C
95. Zhang Y, Azmoun S, Hang A, Zeng J, Eng E, Wildsoet CF. Retinal defocus and form-deprivation induced regional differential gene expression of bone morphogenetic proteins in chick retinal pigment epithelium. J Comp Neurol. (2020) 528:2864–73. doi: 10.1002/cne.24957
96. Zhang Y, Phan E, Wildsoet CF. Retinal defocus and form-deprivation exposure duration affects RPE BMP gene expression. Sci Rep. (2019) 14:7332. doi: 10.1038/s41598-019-43574-z
97. Wu W, Wang Y. Corneal higher-order aberrations of the anterior surface, posterior surface, and total cornea after SMILE. FS-LASIK, and FLEx surgeries. Eye Contact Lens. (2016) 42:358–65. doi: 10.1097/ICL.0000000000000225
98. Zhao X, Zhang L, Ma J, Li M, Zhang J, Zhao X, et al. Comparison of wavefront-guided femtosecond LASIK and optimized SMILE for correction of moderate-to-high astigmatism. J Refract Surg. (2021) 37:166–73. doi: 10.3928/1081597X-20201230-01
99. Ang M, Gatinel D, Reinstein DZ, Mertens E, Alio Del Barrio JL, Alio JL. Refractive surgery beyond 2020. Eye (Lond). (2021) 35:362–82. doi: 10.1038/s41433-020-1096-5
100. Heradio R, Fernandez-Amoros D, Cerrada C, Cobo MJ. Group decision-making based on artificial intelligence: a bibliometric analysis. Mathematics. (2020) 8:1566. doi: 10.3390/math8091566
101. Cao K, Wan Y, Yusufu M, Wang N. Significance of outdoor time for myopia prevention: a systematic review and meta-analysis based on randomized controlled trials. Ophthalmic Res. (2020) 63:97–105. doi: 10.1159/000501937
102. Ashby R, Ohlendorf A, Schaeffel F. The effect of ambient illuminance on the development of deprivation myopia in chicks. Invest Ophthalmol Vis Sci. (2009) 50:5348–54. doi: 10.1167/iovs.09-3419
103. Schaeffel F, Glasser A, Howland HC. Accommodation, refractive error and eye growth in chickens. Vision Res. (1988) 28:639–57. doi: 10.1016/0042-6989(88)90113-7
104. Chia A, Lu QS, Tan D. Five-year clinical trial on atropine for the treatment of myopia 2: myopia control with atropine 0.01% eyedrops. Ophthalmology. (2016) 123:391–9. doi: 10.1016/j.ophtha.2015.07.004
105. Nickla DL, Zhu X, Wallman J. Effects of muscarinic agents on chick choroids in intact eyes and eyecups: evidence for a muscarinic mechanism in choroidal thinning. Ophthalmic Physiol Opt. (2013) 33:245–56. doi: 10.1111/opo.12054
106. Lind GJ, Chew SJ, Marzani D, Wallman J. Muscarinic acetylcholine receptor antagonists inhibit chick scleral chondrocytes. Invest Ophthalmol Vis Sci. (1998) 39:2217–31.
107. Dotan A, Kremer I, Gal-Or O, Livnat T, Zigler A, Bourla D, et al. Scleral cross-linking using riboflavin and ultraviolet-a radiation for prevention of axial myopia in a rabbit model. J Vis Exp. (2016) 3:e53201. doi: 10.3791/53201
108. Choi JA, Han K, Park YM, Park CK. Age-related association of refractive error with intraocular pressure in the Korea National Health and Nutrition Examination Survey. PLoS ONE. (2014) 9:e111879. doi: 10.1371/journal.pone.0111879
109. Yeung AWK. Comparison between Scopus, Web of Science, PubMed and publishers for mislabelled review papers. Curr Sci. (2019) 116:1909–14. doi: 10.18520/cs/v116/i11/1909-1914
Keywords: myopia, public health, bibliometric analysis, global trends, myopia control, refractive surgery, CitNetExplorer
Citation: Shan M, Dong Y, Chen J, Su Q and Wang Y (2022) Global Tendency and Frontiers of Research on Myopia From 1900 to 2020: A Bibliometrics Analysis. Front. Public Health 10:846601. doi: 10.3389/fpubh.2022.846601
Received: 31 December 2021; Accepted: 10 February 2022; Published: 10 March 2022.
Reviewed by:
Copyright © 2022 Shan, Dong, Chen, Su and Wang. This is an open-access article distributed under the terms of the Creative Commons Attribution License (CC BY) . The use, distribution or reproduction in other forums is permitted, provided the original author(s) and the copyright owner(s) are credited and that the original publication in this journal is cited, in accordance with accepted academic practice. No use, distribution or reproduction is permitted which does not comply with these terms.
*Correspondence: Yan Wang, wangyan7143@vip.sina.com
† These authors have contributed equally to this work
Disclaimer: All claims expressed in this article are solely those of the authors and do not necessarily represent those of their affiliated organizations, or those of the publisher, the editors and the reviewers. Any product that may be evaluated in this article or claim that may be made by its manufacturer is not guaranteed or endorsed by the publisher.
Innovation in Eye Care, Research & Education
- See us on facebook
- See us on linkedin
- See us on instagram
Annual Report 2021
• A note from our Chair
• An eye-brain connection: Groundbreaking advancements for neurorehabilitation patients
• Shedding light on rare diseases
• Saving vision with gene therapies
• Biorepository: A new key to precision health
• Eye care at all ages: Bringing vision restoration to pediatric patients
> New center tackles rapidly growing myopia prevalence
• My second chance at sight: A patient’s hopeful journey after optic nerve stroke
• Global impact: Generous donors support global health efforts for cataract blindness
• A hopeful view on eyesight: Grateful patient celebrates Dr. Kuldev Singh’s 30th anniversary in 2022
• Why give?
• Fighting blindness across borders
• Stanford Belize Vision Clinic: Training the next generation of eye care providers
• Training for global care: Ophthalmology resident sets up two eye care programs in the Middle East
• Mentorship leads to new gene therapy discoveries
• 3D bioprinting to eliminate corneal blindness
• Big data to transform patient care
• Inventing a new outlook: Restoring sight with electronic photoreceptors and augmented reality glasses
• Eye care at the microscopic level
• Awards and honors
New center tackles rapidly growing myopia prevalence
The prevalence of myopia , or nearsightedness , continues to grow, affecting about 42% of the entire American population. Outside of the U.S., urban areas of Singapore, China, Taiwan, Hong Kong, Japan and Korea report upwards of 80 to 90% of high school graduates are myopic.
Myopia is a disorder where the eye grows longer than average, causing light to focus in front of the retina instead of on the retina, leading to blurred vision. Fortunately, most cases of myopia are considered mild or moderate and glasses, contacts, or refractive surgery can provide clear vision. More progressive or severe cases, however, carry significantly higher risk of retinal and optic nerve degeneration and vision loss that cannot be so easily managed.
Recognizing that myopia impacts people of all ages, a multidisciplinary faculty team at the Byers Eye Institute at Stanford established the groundbreaking Myopia Center of Excellence to address offering the latest therapies, opportunities for laboratory and clinical research, and new avenues of education outreach.
“While still in its early stages, this collaborative center already meets a great need, because myopia spans across multiple subspecialties within ophthalmology and optometry,” Tawna Roberts, OD, PhD, assistant professor of ophthalmology, said. “The goal is to slow the progression of myopia during childhood and also later in life, as patients with myopia are at a higher risk of developing glaucoma, cataracts, retinal detachments, and other problems that lead to vision loss.”
Roberts has played a key role in establishing the center along with Ann Shue, MD, clinical assistant professor of ophthalmology and a pediatric ophthalmology and glaucoma specialist, who said the specific cause for myopia is unclear.
“We know the shape of the eyeball and cornea, genetics, and environmental factors such as reduced sunlight and excessive screen time can all play a role, but there is still a lot to learn,” Shue said.
In addition to Roberts and Shue, Robert Chang, MD, associate professor of ophthalmology, and Steven Sanislo, MD, clinical professor of ophthalmology, treat adults with visual and ocular complications from myopia while Katie Warner, OD, clinical assistant professor of ophthalmology, joins Roberts in treating pediatric patients.
“As myopic eyes elongate it causes stretching to the retina and increases the risk for retinal tears,” Sanislo said. “Severely myopic eyes can also develop myopic macular degeneration which can lead to serious vision disability. Therapies that reduce the elongation of the eye will benefit myopia patients of all ages.”
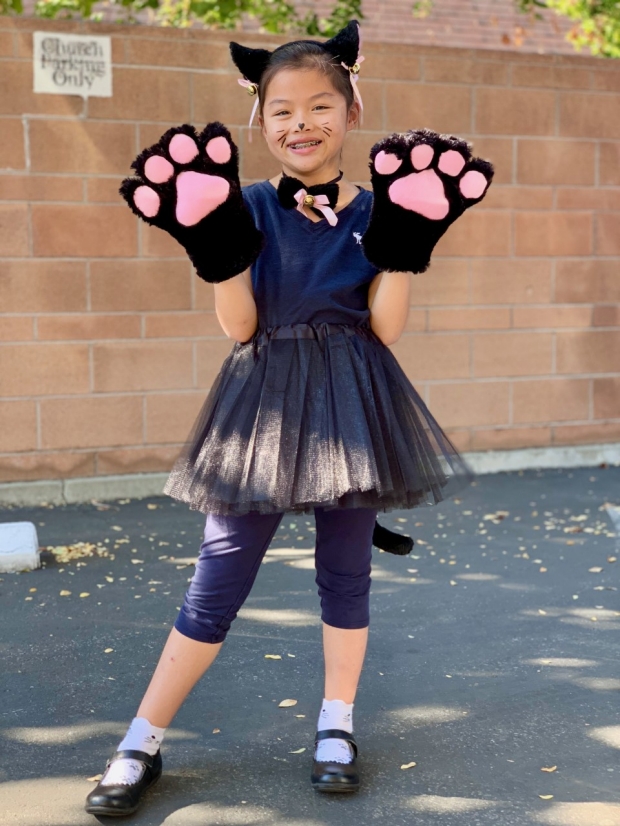
Annabelle dresses up as a cat on Halloween.
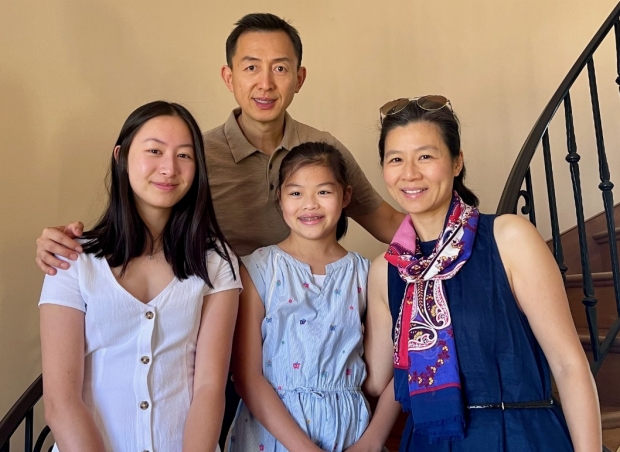
Annabelle and her family in August 2021.
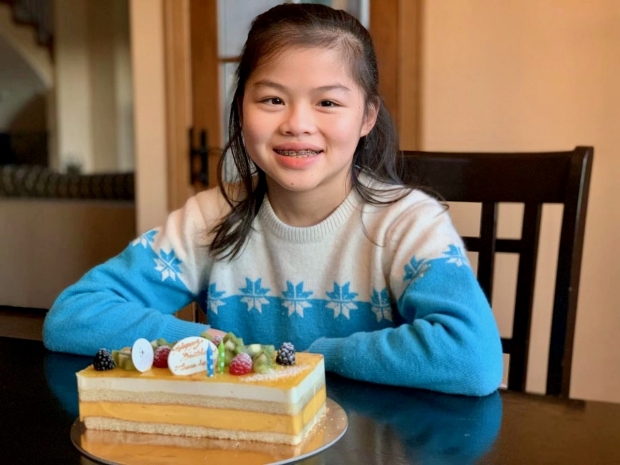
Annabelle celebrates her 11th birthday.
Through a patient’s eyes
One of the first patients receiving treatment in the new Myopia Center is 11-year-old Annabelle Yao , who has been a lifelong patient at Stanford. A few months after Annabelle was born, her parents Weijing Shen and Frank Yao noticed her skin began developing an unhealthy pale color. Concerned, they took Annabelle to Lucile Packard Children’s Hospital Stanford , where she was quickly sent to the Pediatric Emergency Department at Stanford Hospital .
They discovered both of Annabelle’s kidneys had failed, causing toxins to build up in the body, leading to skin color changes and a higher risk of complications in her brain and eyes. Until Annabelle could receive a kidney transplant, she was put on dialysis, a process that takes over the function of the kidney by removing waste and extra fluid from the blood.
Annabelle’s health made tremendous improvement since birth and she started being seen every three months by a team of specialists.
“Around the age of six, we began taking Annabelle to Byers to have her vision monitored when we noticed she had strabismus—her eyes were looking in different directions,” Weijing said.
After doing a year of school virtually during COVID-19, Annabelle’s parents also noticed that her nearsightedness was worsening at a much faster rate. Annabelle was previously given a glasses prescription, but the glasses did not slow down the progression of her myopia. Roberts recommended two new treatments to prevent Annabelle’s myopia from worsening so quickly: multifocal contact lenses, which provide clear vision while simultaneously slowing eye growth, and low-dose atropine eye drops. Annabelle’s parents opted for the latter.
“Atropine is a nervous system blocker often used for patients with slow heart rate or stomach complications,” Roberts said. “Historically, it has been used in the eyes primarily to treat eye pain from inflammation and to treat amblyopia. But recent research and clinical trials have shown that it can also significantly slow down the growth of the eye, so we use it for myopia.”
Roberts noted that while it is still unknown how to prevent myopia entirely, the drops can help keep Annabelle’s myopia from being as high in her adult years as it would be without any intervention.
Throughout all her health complications, Annabelle has remained as her parents describe “cheerful, positive, and independent.”
“We often joke that Annabelle’s favorite saying is ‘I can do that’ because she is very independent,” Frank said. “Even at a young age, she has a desire to continuously overcome any obstacle she faces, which has really been an encouragement to both us and her older sister, Sophia.”
Weijing and Frank relate Annabelle’s early years when she made a miraculous recovery from her kidney failure, to now making many friends at school and taking up her newest hobby of dancing.
“While this has been a long journey for Annabelle and our family, we have received tremendous support from the entire Stanford team,” Frank said. “We are grateful for the exceptional patient care that Dr. Roberts has provided Annabelle in this entire process and cannot thank her enough.”
By KATHRYN SILL
Kathryn Sill is a web and communications specialist for the Byers Eye Institute in the Department of Ophthalmology, at Stanford University School of Medicine. Email her at [email protected] .

Screen time linked to risk of myopia in young people
High levels of exposure can increase risk of short-sightedness by up to 80%.
A new study published in one of the world's leading medical journals has revealed a link between screen time and higher risk and severity of myopia, or short-sightedness, in children and young adults.
The open-access research, published this week in The Lancet Digital Health, was undertaken by researchers and eye health experts from Singapore, Australia, China and the UK, including Professor Rupert Bourne from Anglia Ruskin University (ARU). The authors examined more than 3,000 studies investigating smart device exposure and myopia in children and young adults aged between 3 months old and 33 years old.
After analysing and statistically combining the available studies, the authors revealed that high levels of smart device screen time, such as looking at a mobile phone, is associated with around a 30% higher risk of myopia and, when combined with excessive computer use, that risk rose to around 80%.
The research comes as millions of children around the world have spent substantial time using remote learning methods following the closure of schools due to the COVID-19 pandemic.
Professor Bourne, Professor of Ophthalmology in the Vision and Eye Research Institute at Anglia Ruskin University (ARU), said: "Around half the global population is expected to have myopia by 2050, so it is a health concern that is escalating quickly. Our study is the most comprehensive yet on this issue and shows a potential link between screen time and myopia in young people.
"This research comes at a time when our children have been spending more time than ever looking at screens for long periods, due to school closures, and it is clear that urgent research is needed to further understand how exposure to digital devices can affect our eyes and vision. We also know that people underestimate their own screen time, so future studies should use objective measures to capture this information."
- Children's Health
- Child Psychology
- Child Development
- Educational Psychology
- Educational Technology
- Mobile Computing
- Communications
- Hyperactivity
- Adult attention-deficit disorder
- Growth hormone treatment
- Spinal muscular atrophy
- Methylphenidate
- Sex education
Story Source:
Materials provided by Anglia Ruskin University . Note: Content may be edited for style and length.
Journal Reference :
- Joshua Foreman, Arief Tjitra Salim, Anitha Praveen, Dwight Fonseka, Daniel Shu Wei Ting, Ming Guang He, Rupert R A Bourne, Jonathan Crowston, Tien Y Wong, Mohamed Dirani. Association between digital smart device use and myopia: a systematic review and meta-analysis . The Lancet Digital Health , 2021; DOI: 10.1016/S2589-7500(21)00135-7
Cite This Page :
Explore More
- Fossil Frogs Share Their Skincare Secrets
- Fussy Eater? Most Parents Play Short Order Cook
- Precise Time Measurement: Superradiant Atoms
- Artificial Cells That Act Like Living Cells
- Affordable and Targeted Anticancer Agent
- This Alloy Is Kinky
- Giant Galactic Explosion: Galaxy Pollution
- Flare Erupting Around a Black Hole
- Two Species Interbreeding Created New Butterfly
- Warming Antarctic Deep-Sea and Sea Level Rise
Trending Topics
Strange & offbeat.
Multiple Factors Causing Myopia and the Possible Treatments: A Mini Review
Affiliations.
- 1 Laboratory of Photobiology, Keio University School of Medicine, Tokyo, Japan.
- 2 Department of Ophthalmology, Keio University School of Medicine, Tokyo, Japan.
- 3 Tsubota Laboratory, Inc., Tokyo, Japan.
- PMID: 35619815
- PMCID: PMC9127355
- DOI: 10.3389/fpubh.2022.897600
The myopia epidemic has become a global public health problem. Although myopia is progressing worldwide, the recent coronavirus infections 2019 (COVID-19) outbreak has spurred myopia progression. The current evidence-based treatments for humans are atropine eye drops, optical treatment with defocus, use of orthokeratology, extending proximity working distance, pausing from near work every half hour and increased time outside the home. Studies on myopia using animal models have been conducted for more than 40 years. In recent years, new mechanisms of myopia suppression have been revealed from animal experiments such as inflammation control, intraocular pressure control, light control, and the activity of early growth response protein 1 control. This mini-review provides a summary of the scientific evidence currently available on the control of myopia, and the possible treatments mitigating myopia.
Keywords: Asian; anthropometrics; axial length (AL); myopia; treatments.
Copyright © 2022 Shinojima, Negishi, Tsubota and Kurihara.
Publication types
- Research Support, Non-U.S. Gov't
- Atropine / therapeutic use
- Disease Progression
- Myopia* / epidemiology
- Myopia* / therapy
- Ophthalmic Solutions
- Alzheimer's disease & dementia
- Arthritis & Rheumatism
- Attention deficit disorders
- Autism spectrum disorders
- Biomedical technology
- Diseases, Conditions, Syndromes
- Endocrinology & Metabolism
- Gastroenterology
- Gerontology & Geriatrics
- Health informatics
- Inflammatory disorders
- Medical economics
- Medical research
- Medications
- Neuroscience
- Obstetrics & gynaecology
- Oncology & Cancer
- Ophthalmology
- Overweight & Obesity
- Parkinson's & Movement disorders
- Psychology & Psychiatry
- Radiology & Imaging
- Sleep disorders
- Sports medicine & Kinesiology
- Vaccination
- Breast cancer
- Cardiovascular disease
- Chronic obstructive pulmonary disease
- Colon cancer
- Coronary artery disease
- Heart attack
- Heart disease
- High blood pressure
- Kidney disease
- Lung cancer
- Multiple sclerosis
- Myocardial infarction
- Ovarian cancer
- Post traumatic stress disorder
- Rheumatoid arthritis
- Schizophrenia
- Skin cancer
- Type 2 diabetes
- Full List »
share this!
November 21, 2023
This article has been reviewed according to Science X's editorial process and policies . Editors have highlighted the following attributes while ensuring the content's credibility:
fact-checked
trusted source
The myopia epidemic: Blame computers not phones for short-sightedness, researcher says
by University of Western Australia
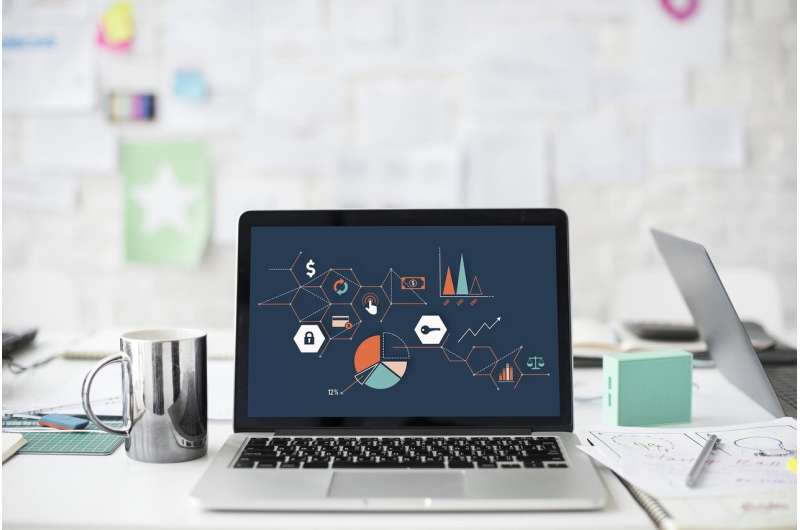
A new study from The University of Western Australia has found the world is experiencing a myopia (short-sightedness) epidemic, and the main cause is computer screens rather than mobile phones or tablets.
Senior research fellow Samantha Sze-Yee Lee from the Lions Eye Institute used data from the Raine Study collected from 600 young adults to discern the types of digital screens that contributed to the development of myopia.
Dr. Lee found that short-sightedness worsened faster in those who reported six or more hours per day of computer usage compared to those with low computer use, while time spent on mobile phones did not have any effect.
"The reason for this difference may be due to a phenomenon called 'peripheral defocus,'" Dr. Lee said.
"When you look at your mobile phone , everywhere in our peripheral vision, with the exception of the small phone screen, is further away and relatively blurred."
"The brain registers things are generally far away, and there is no need for the eye to become more short-sighted."
"When you focus on a large screen such as a desktop computer, more of our peripheral vision is taken by the screen."
"The brain sees that more short-distance work is involved, triggering the eyes to become more short-sighted."
Myopia is associated with an increased risk of future eye problems, such as glaucoma and retinal degeneration , so understanding how our modern world affects our risk is important.
"In this day and age, it is almost impossible to avoid digital screens," Dr. Lee said.
"Mobile phones can easily be used outdoors, as opposed to laptop and desktop computers, and spending more time outdoors is known to be protective against myopia."
"It is hoped the findings will help scientists develop techniques to mitigate the detrimental impact of computer screens on eyesight."
Based in Perth, Western Australia, the Raine Study is one of the largest and longest-running studies of human health from pregnancy through childhood, adolescence, and adulthood to be carried out anywhere in the world.
Explore further
Feedback to editors

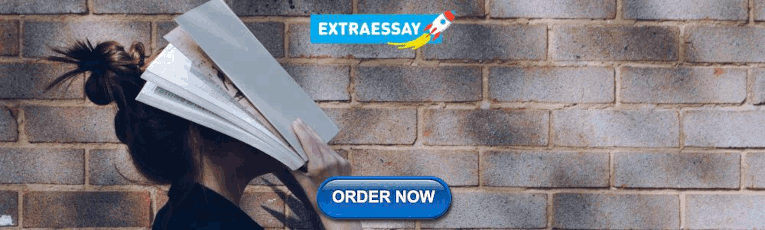
Study highlights increased risk of second cancers among breast cancer survivors
2 hours ago

Opioids during pregnancy not linked to substantially increased risk of psychiatric disorders in children
3 hours ago

Research identifies pitfalls and opportunities for generative AI in patient messaging systems

A closed-loop drug-delivery system could improve chemotherapy
5 hours ago

It's easier now to treat opioid addiction with medication—but use has changed little, study finds

Solving the riddle of the sphingolipids in coronary artery disease

New AI technology estimates brain age using low-cost EEG device

Alteration of brain network condition could predict painful vaso-occlusive crisis in patients with sickle cell disease
7 hours ago

Trials reveal that internet-based conversations help sustain brain function in older adults

Study finds that a dash of exercise can help students focus and enjoy university lectures
Related stories.

Screen time linked to risk of myopia in young people
Oct 8, 2021

Researchers tackle mystery of short-sightedness increasing in children
Jun 16, 2021
Short-sightedness in kids was rising long before they took to the screens
Jan 7, 2020

Myopia linked to poor sleep and screen time
May 24, 2021

Hidden in plain sight: How the COVID-19 pandemic is damaging children's vision
Apr 28, 2021

Myopia progression may continue in young adults into late 20s
Jan 12, 2022
Recommended for you

Expert reviews the current state of retinoblastoma research
Apr 22, 2024

Analyzing the progression in retinal thickness could predict cognitive progression in Parkinson's patients
Apr 19, 2024

Potential modifier gene identified as cause of ciliary pathology in retinitis pigmentosa patient

No negative impact from prolonged eye patching on child's development or family stress levels
Apr 18, 2024

AI beats doctors in accurately assessing eye problems
Apr 17, 2024

Genetic variants found in two types of strabismus, sparking hope for future treatment of eye condition
Let us know if there is a problem with our content.
Use this form if you have come across a typo, inaccuracy or would like to send an edit request for the content on this page. For general inquiries, please use our contact form . For general feedback, use the public comments section below (please adhere to guidelines ).
Please select the most appropriate category to facilitate processing of your request
Thank you for taking time to provide your feedback to the editors.
Your feedback is important to us. However, we do not guarantee individual replies due to the high volume of messages.
E-mail the story
Your email address is used only to let the recipient know who sent the email. Neither your address nor the recipient's address will be used for any other purpose. The information you enter will appear in your e-mail message and is not retained by Medical Xpress in any form.
Newsletter sign up
Get weekly and/or daily updates delivered to your inbox. You can unsubscribe at any time and we'll never share your details to third parties.
More information Privacy policy
Donate and enjoy an ad-free experience
We keep our content available to everyone. Consider supporting Science X's mission by getting a premium account.
E-mail newsletter

An official website of the United States government
The .gov means it’s official. Federal government websites often end in .gov or .mil. Before sharing sensitive information, make sure you’re on a federal government site.
The site is secure. The https:// ensures that you are connecting to the official website and that any information you provide is encrypted and transmitted securely.
- Publications
- Account settings
Preview improvements coming to the PMC website in October 2024. Learn More or Try it out now .
- Advanced Search
- Journal List
- Lippincott Open Access

A Review of Current Concepts of the Etiology and Treatment of Myopia
Myopia occurs in more than 50% of the population in many industrialized countries and is expected to increase; complications associated with axial elongation from myopia are the sixth leading cause of blindness. Thus, understanding its etiology, epidemiology, and the results of various treatment regiments may modify current care and result in a reduction in morbidity from progressive myopia. This rapid increase cannot be explained by genetics alone. Current animal and human research demonstrates that myopia development is a result of the interplay between genetic and the environmental factors. The prevalence of myopia is higher in individuals whose both parents are myopic, suggesting that genetic factors are clearly involved in myopia development. At the same time, population studies suggest that development of myopia is associated with education and the amount time spent doing near work; hence, activities increase the exposure to optical blur. Recently, there has been an increase in efforts to slow the progression of myopia because of its relationship to the development of serious pathological conditions such as macular degeneration, retinal detachments, glaucoma, and cataracts. We reviewed meta-analysis and other of current treatments that include: atropine, progressive addition spectacle lenses, orthokeratology, and multifocal contact lenses.
Myopia is a common and yet perplexing ocular disorder. Once viewed as a benign refractive condition, today myopia, even at low levels, is associated with increased risk for numerous ocular diseases. 1 Researchers have reported on the myopia epidemic, which is occurring worldwide. 2 Although the exact etiology of myopia remains elusive, it appears to have both genetic and environmental components, 3 making prevention and treatment both challenging and individualized. Stopping the progression of myopia has the potential to positively affect quality of life and ocular health. Popular control options today include progressive addition lenses (PAL), topical atropine, orthokeratology (OK) lenses, and multifocal contact lenses. The intent of this review is to provide the most current information about myopia etiology and treatment strategies with the goal that ocular health may be preserved.
PREVELANCE AND ETIOLOGY OF MYOPIA
Myopia is the most common ocular disorder worldwide. 4 The prevalence of myopia in the United States has increased from 25% to 44% between 1972 and 2004. 5 – 7 In urban communities in Asia, the prevalence is greater than 80%. 8 , 9 The prevalence is much lower in underdeveloped areas in the world such as Sherpa in Nepal. 10
The economic burden of eye diseases is approximately $139 billion in the United States, with nearly $16 billion spent on myopia correction alone. 5 , 6 , 11 Myopia represents a major risk factor for a number of other ocular pathologies such as cataract, glaucoma, retinal detachment, and myopic maculopathy, which is comparable to the risks associated with hypertension for stroke and myocardial infarction. 1 , 12 Taking into account pathological complications of myopia and other serious pathologies associated with the disease, myopia not only negatively affects self-perception, job/activity choices, and ocular health, 13 – 15 but also represents one of the leading causes of blindness in the world. 16 The yearly incidence of retinal detachments is 0.015% in patients with less than 4.74 diopters (D) myopia and it increases to 0.07% in myopia greater than 5 D and 3.2% myopia greater than 6 D. 17 , 18 Myopic patients also have great risk of developing macular choroidal neovascularization, that is, 2X for patients with 1 D to 2 D of myopia; 4X with 3 D to 4 D of myopia; and 9X for 5 to 6 D of myopia. 19 It is estimated that 4.8 billion people (one half of the world's population) will be affected by myopia by 2050. 20 A recent study reported that 10% of Asian high school students have high myopia, which increases the risk for future retinal disease. 21
Historically, some eye care professionals have believed that myopia is a hereditary anomaly, whereas others have believed that myopia is environmentally induced. However, human and animal studies conducted over the last four decades suggest that development of myopia is controlled by both environmental and genetic factors. 22 – 25
Human population studies have revealed that environmental factors, such as near work and reading, play an important role in the development of myopia. 26 – 32 However, this is not without some controversy. 33 Zylbermann et al. 34 analyzed the incidence of myopia in two groups: Orthodox Jewish students (male and female, where males, unlike females, spent the majority of the day reading) and secular Jewish students (male and female, where both men and women spent less time reading than the male students in the orthodox Jewish schools). They found that the Orthodox Jewish male students had a much higher incidence and degree of myopia as compared to the other three groups of students. This finding suggests that reading was the factor that caused myopia.
In addition, there are a number of epidemiological studies that show that myopia is more common in urban areas, among professionals, educated patients, computer users, university students, and associated with increased intelligence. 35 – 43 There is evidence that the intensity of reading may be more important than the actual time spent reading. 44 Myopia is also increased in individuals who perform tasks requiring increased use of eyes such as microscopists. 45 These and other findings of the association between near work and myopia were complemented by the observations that near work and reading are associated with the lag of accommodation, that is, insufficiently strong accommodative response to near objects, which places the plane of best focus behind the retina (hyperopic defocus) when the subject performs near work tasks. 46 , 47 This observation led to the theory that the optical blur such as produced by the lag of accommodation may be the signal that drives excessive eye growth and causes myopia. 27 , 46 , 48 – 51 This theory is supported by the numerous animal studies, which have found that degradation of visual input using either diffusers or negative lenses causes excessive eye growth and myopia in species as diverse as fish, chickens, tree shrews, monkeys, guinea pigs, and mice. 52 – 67
Wiesel and Raviola were the first to induce experimental myopia in an animal model. They placed a translucent screen over a monkey's eye causing it to become severely myopic; however, when total occlusion was used instead, there was no change in the length of the eye. 61 , 68 Thus, stimulation of the retina with a blurred image results in alteration of the growth signals within the eye. Numerous studies performed in animals using both positive and negative lenses have demonstrated that the eye will change its axial length (AL) to accommodate for the lens placed in front of the eye. 69 – 72 This change is reversible because some animals are able to recover when the visual stimuli is removed. 73 This change in AL occurred even if the optic nerve was severed. 74 It occurred in half the eye, if only half the eye was exposed to blur using a diffuser or plus or minus lenses. 75 The fact that the eye responds to local blur with local changes even when the optic nerve is severed demonstrates that the signaling cascade regulating refractive eye development is within the eye itself and does not require a feedback from the brain (Fig. (Fig.1). 1 ). Rada et al. 76 reported that the retina provides remodeling signals to the sclera by which the eye alters its shape to place an image on the retina, that is, emmetropization.

Regional blur causes axial elongation. Regional retinal blur created in half the retina causes regional elongation of the eye. This occurs even when the optic nerve is cut, but will not occur if atropine is injected into the eye. The eye recognizes the direction of the blur, that is, plus or minus lenses and the region of retinal blur. Reprinted with permission from Cooper J, Schulman E, Jamal N. Current status on the development and treatment of myopia. Optometry 2012;83:179–99.
Smith et al. experimentally asked the most important question. Does the eye respond to foveal blur, peripheral blur, or equally to both? 77 , 78 Smith created a series of lenses in which the center was minus and the periphery was plus and lenses in which the center was plus and the periphery was minus. In both cases, the length of the eye changed in response to the peripheral lens power. For instance if the central lens was plus and the peripheral part of the lens was minus, the eye elongated. Lastly, Smith et al. 79 ablated the macula of a number of monkeys. In this instance, the eyes still changed their AL in response to the lens power. These studies suggest that defocus information is summed up across the entire surface of the retina and the integrated signal regulates the growth of the eye (Fig. (Fig.2 2 ). 74 , 78 , 80 Many clinicians and researchers believe that these animal studies have a direct relationship to the development of axial elongation or myopia and they have suggested that treatment should be based on these models. 2 , 78 , 81

Image shells on the retina. Once the eye elongates in myopia, optical images from spherical lenses no longer fall on the retinal plane. The peripheral images are out of focus falling on a plane behind the retina. It is thought that the relative hyperopic error created is the stimulus for axial elongation. Current optical treatments move the peripheral focus in front of the retina.
Myopia seems to progress the most between ages 8 and 15 (Caucasians X=0.6 D/year. and Asians X=0.7 D/year.) and then begins to slow down. 82 – 84 Mutti et al. 85 reported that in a large cohort of subjects, which developed myopia, a year or two before the onset of myopia, hyperopic defocus developed in the periphery of the eye along the horizontal meridian. This relative hyperopia is believed to be growth signal. If this hyperopic, defocus is altered optically to create myopic defocus by using plus power in the periphery; according to this theory, a stop signal is created. This is the basis for most optical treatments. 77
Myopia increases the most during the winter and the least during the summer months. 86 , 87 It is unknown if this is because of increased school work, decreased sunlight, or decreased time outside. In previous generations, myopic progression was assumed to end at age 18. 88 However, that has changed since more students have entered graduate school followed by jobs requiring 8 hr of sustained computer work. 43 This conjecture was recently studied in a cohort of post-university graduates with a mean age of 35. 89 Myopia was found to progress in approximately 10% of the cohort who spent a lot of time in front of computers. Those subjects who did not spend time in front of computers did not progress as much. In addition, Bullimore et al. 90 reported that 21% of contact wearers between the ages of 20 and 40 years of age progressed at least 1 D over 5-year period of follow-up.
All these human and animal studies strongly suggest that environmental factors play a an important role in the development and progression of myopia; however, human population studies suggest the contribution of genetic factors accounts for at least 70% of variance in refraction. 91 – 95 It is clear that the incidence of myopia increases when both parents have myopia. 38 , 96 Numerous studies have shown that the refractive error of the parents is the most important predictor of the development of myopia. 97 , 98 Strong support also comes from studies comparing monozygotic 99 and dizygotic twins. 91 , 100 , 101 The refractive error is thought to be influenced by multiple interacting genes. 91 – 93 Multiple chromosomal loci, which are linked to human myopia, have been identified. 23 , 102 – 128 However, myopia appears to be a rather heterogeneous disease because the genetic loci and genetic variants associated with myopia in different families and ethnic groups are often distinct. 23 , 25 , 102 – 127 Considering that complex quantitative traits such as myopia are often controlled by dozens or even hundreds of chromosomal loci, 129 and that the identified chromosomal loci could account for less than 25% of myopia cases, 107 only a small fraction of chromosomal regions that control refractive eye development has been identified.
Thus, both environmental and genetics factors have been shown to contribute to myopia development 22 – 25 ; however, it was not clear whether these factors act independently or if there was some form of interaction. Recent work by Tkatchenko et al. 3 has helped consolidate the dichotomy of views related to the etiology of myopia, that is, genes versus environment. These authors studied a three-way interaction between age, time spent reading, and genetic variation at APLP2 gene locus. It was found that children who spent a “high” amount of time reading and who had the myopic version of APLP2 gene were 5 times more likely to develop myopia compared to those children who spent “low” amount of time reading. On the contrary, children who carried a normal version of APLP2 did not develop myopia even if they were exposed to high levels of reading. To confirm the human findings, they studied refractive eye development in APLP2 knockout mice and found similar interaction between APLP2 and visual experience in mice. This study demonstrated for the first time gene–environment interaction in myopia development and suggested that genetic background of an individual determines the impact of environmental factors on refractive eye development.
TREATMENT OF MYOPIA
The incidence of retinal detachment and macular degeneration increases logarithmically above 2 diopters of myopia (Fig. (Fig.3 3 ). 1 To put this in perspective, keeping myopia at −1.00 versus −3.00 D reduces the risk of macular degeneration by 4 times and retinal detachment by 3 times. Brennan 130 reported that reducing progression by 33% would result in a 73% reduction in myopia progression above 5 D; if the reduction rate improved to 50%, then there would be 90% reduction of myopia above 5 D. Thus, myopia control becomes an increasingly important issue because recent environmental changes have not only resulted in a sharp increase in the incidence of myopia worldwide, but caused an increase in the age of progression and the ultimate increase in the magnitude of the refractive error. In our opinion, patients should be presented with the current risks and benefits of the various treatment options available for myopia control.

Risk of ocular disease with increased myopia. It is readily apparent that the risk of retinal detachment and macular degeneration increases logarithmically with the increase of acquired myopia.139 The risk begins with as little as 1.00 D of myopia. Reprinted with permission from Flitcroft DI. The complex interactions of retinal, optical and environmental factors in myopia aetiology. Prog Retin Eye Res 2012;31:622–60.
Animal and human studies have important practical consequences for the treatment of myopia. They specifically suggest that reducing lag of accommodation, reducing both central and peripheral defocus, and blocking myopiagenic signaling in the eye should slow the progression of myopia. Considering that the information about signaling pathways underlying myopia development is limited, the currently considered treatment modalities for control of myopic progression include optical correction such as bifocal spectacle lenses, progressive addition spectacle lenses, under-correction, OK, multifocal contact lenses, and increased exposure to outdoor activities, with the notable exception of atropine which has been shown to block myopiagenic signaling albeit with some uncomfortable side effects. 81
Bifocal spectacle lenses were the first to be used extensively to control myopia progression. The lenses were prescribed based on the assumption that myopia was a response to prolonged accommodation producing optical blur. 51 , 81 , 131 , 132 There have been a number of retrospective studies, which showed that bifocals and PALs slow the progression of myopia. 133 – 135 On average, these studies suggested that myopia was slowed by 40%. However, these studies had some issues with experimental design, for example, they were retrospective, unmasked, etc. The COMET (The Correction of Myopia Evaluation Trial) study was designed to determine if a +2.00 D PAL slowed the progression of myopia as compared to a single-vision (SV) full correcting spectacle lens. 136 This NIH/NEI prospective, multicenter clinical trial demonstrated that in the first year, PALs slowed the progression of myopia by 20%. However, the effect was significantly reduced in years 2 to 4. The net reduction was 0.2 D, which was clinically insignificant but reached statistical significance. The progressive lenses were the most effective when both parents were myopic, there was a large lag of accommodation and/or the children had esophoria at near. 51 , 137
Recently, Cheng et al. 138 studied the use of high fitting executive bifocal spectacle lenses with base-in prism as compared to SV lenses in a group of Canadian Asians. The experimental lens slowed the progression of myopia by 40%. However, this study was not masked and was not double-blinded. In 2011, Shi-Ming Li et al. performed a meta-analysis of 9 clinical trials in which powers of PALs ranged from +1.5 to +2.0 D and found that PALs slowed myopic progression by 0.25 D/year as compared to SV lenses. The effect was greater in Asian children as compared to Caucasians and also greater in children who had a higher level of myopia at baseline and who progressed at a more rapid rate (Fig. (Fig.4 4 ). 139

Meta-analysis of progressives and bifocal spectacle lenses. Meta-analysis 139 of 9 clinical trials in which progressive additional and bifocal spectacle lenses (MFL) are compared with single-vision lenses (SVL) using spherical equivalent (A) and axial length (B). Mean difference between SVL and MFL was 0.25 D per year and in those that reported axial length changes, the difference was 0.012 mm. 137 , 244 – 251 The benefit of MFL was greater in Asian versus white children (0.32 D vs. 0.10 D) and/or those that initially had a higher baseline refraction. (Less than 3 D at baseline = 0.16 D vs. greater than 3 D at baseline 0.39 D). It should be noted that these findings were not replicated in an analysis of 16 treatment protocols for myopia. 243 Reprinted with permission from Li SM, Ji YZ, Wu SS, et al. Multifocal versus school-age children: a meta-analysis. Surv Ophthalmol 2011;56:451–60.
In a novel experiment, spectacle lenses, which were designed to reduce peripheral hyperopic defocus, were evaluated to determine their effect on the progression of myopia in Chinese children aged 6 to 16 years. 140 The authors reported that none of the spectacle lenses had any significant effect in slowing the progression of myopia. Failure to achieve a significant result is believed to be related to the constant changing of eye position when viewing through the lenses.
Historically, many eye care professionals have under-corrected myopia in the belief that the myopic progression will slow down as a result of reduced accommodation. However, with today's knowledge that blur affects the ability of the eye to become emmetropic, this is intuitively incorrect. Two recent studies have demonstrated that under-correction actually results in mild acceleration of myopia progression. 141 , 142 Thus, under-correction should not be used to slow myopic progression.
Contact Lenses
For years, it was believed that gas permeable contact lenses slowed the progression of myopia. However, it should be remembered that gas permeable contacts typically are prescribed when myopia begins to slow down (12 and older) and that these contact lenses flatten the cornea. In a number of well-controlled clinical trials, it has been shown that neither conventional soft nor gas permeable contact lenses alter the progression of myopia. 143 , 144
In 2003, Reim et al. 145 performed a retrospective study of 253 children (ages 6–18) on the ability of OK to slow the progression of myopia. He reported that the rate of progression was slowed from 0.5 to 0.13 D/year. Subsequently, there have been a number of prospective clinical trials, which have demonstrated that OK tends to slow the progression of myopia by 40% using AL measurements and wash-out cycloplegic measurements. 146 – 154 Two separate meta-analyses of these studies, which included 435 patients across 7 studies, demonstrated support for OK's ability to slow myopic progression. 155 , 156 All 7 studies reported AL changes after 2 years, whereas 2 studies reported vitreous chamber depth changes. The pooled estimates indicated change in AL in the OK group. Myopic progression was reduced by approximately 45% (Fig. (Fig.5 5 ).

Meta-analysis of orthokeratology. Meta-analysis of 7 OK studies 155 , 156 was performed, which included 435 subjects who were aged between 6 and 16 years. 148 , 150 , 151 , 241 , 252 – 254 Meta analysis found a mean difference between controls and OK patients of 0.26 mm over 2 years. This is a 40% reduction in the progression of myopia. Reprinted with permission from Si JK, Tang K, Bi HS, Guo DD, Guo JG, Wang XR. Orthokeratology for myopia control: A meta-analysis. Optom Vis Sci 2015.
Swarbrick et al. 152 studied 26 myopic children (11–17 years of age) of East Asian ethnicity using a crossover design study. All of the children were fitted with an overnight OK lens in one eye and a conventional rigid gas-permeable (RGP) lens for daytime wear in the contralateral eye. After 6 months, the lens–eye combinations were reversed and lens wear was continued for another 6 months. After 6 months of lens wear, the average AL of the RGP eye had increased by an average of 0.04 mm, whereas the OK eye showed no change. After the second 6-month phase of lens wear, the OK eye showed no change from baseline in AL, whereas the conventional RGP eye demonstrated a significant increase in mean AL, that is, 0.09 mm. In summary, the conventional RGP lens-wearing eye showed progressive AL growth (myopic progression) throughout the study while the OK eye did not.
There have been two other OK studies that have some reasonable long-term data (5 years and 7 years) demonstrating the myopia control effect of OK. 153 , 154 Orthokeratology provides patients with a “wow” factor and the elimination of daily wearing of contact lenses or glasses. This is particularly beneficial for more athletic children. Visual acuity is quite good with the majority achieving 20/20 and over 90% achieving 20/30. 157
Many eye care professionals believe that the change in the curvature of the cornea is achieved by mechanical flattening of the cornea. However, there is a strong evidence that the change in refraction is achieved by horizontal movement of epithelial cells that occurs from the reverse pressure made from the seal created in the mid-periphery bearing area of the lens. 158 , 159 Proper fitting requires a 20-μm postlens/precorneal tear film. The most significant complaints found with OK are halos secondary to the spherical aberration, which also reduces visual acuity and contrast sensitivity, or discomfort from the lenses. 160
The true risk of infection with OK is unknown. 161 Any risk from infection in a voluntary treatment program that involves children must be weighed against the potential benefit of future reduction of ocular complications such as retinal detachment and macular degeneration. The best estimate of the risk of microbial keratitis (MK) from OK is slightly less than from extended wear of contact lenses. The overall rate is 7.7 per 10,000 years of wear. 162 This compares to 1.4 per 10,000 patient years of wear in nonwearers, 11.9 per 10,000 patient years of wear in silicone hydrogel daily wearers, and 20 per 10,000 patient years of wear in soft contact lens extended wear. 163 This is not a surprise because the lenses are on for a maximum of 8 to 10 hr per day compared to 24 hr for extended wear lenses, the lenses are more oxygen permeable than soft lenses, and the surface of the lens is smoother or slipperier than soft lenses, so that a biofilm does not stick to the lens as easily. The incidence of MK is higher in children than adults. 164 The low incidence should not be dismissed; however, the majority of infections can be handled with aggressive antimicrobial therapy. The rare cases that result from Acanthamoeba or Fusarium infection often result in an avoidable damage to the cornea. 165 Thus, proper hygiene and cleaning is imperative. These lenses, like all other contact lenses, should never be soaked in tap water. Because of the nature of the wearing of the lenses at night, there is greater opportunity for the parents to supervise the wearing of these lenses versus regular soft lenses.
The largest effect from OK is achieved in children who have moderate myopia (between 1.25 and 4.0 diopters) and have larger pupils. It is more difficult to get good results with lower (presumed to be because of the lower mid-periphery plus induced) or higher myopia (inability to achieve targeted prescription). 154 , 166 – 168 Infrequent corneal infiltrates can be minimized by the use of hydrogen-peroxide solutions and mild flattening of the landing zone of the lenses. Published dropout rates are around 20%; however, the children who stay in the program are happier than children fit with traditional contact lenses. 148
Cho and Cheung 169 evaluated the rebound effect when OK lenses were discontinued by comparing the AL in two groups. Group 1 wore OK lenses for 24 months, discontinued lens wear for 7 months, wore SV spectacles (phase I), and then resumed OK lens wear for another 7 months. Group 2 was a control group, which wore spectacles. Stopping OK lens wear resulted in a more rapid increase in AL as compared to those wearing spectacles during the initial 2-year myopia control study (rebound effect). Axial elongation slowed again when OK was resumed. This study suggests that there is a rebound effect with OK lenses.
Recently, there has been renewed increased interest in the use of soft lenses to create similar optics as OK. 170 – 176 To design an optically similar lens, one would need to manufacture a multifocal lens with a distance center. Smaller optic zones are preferable because the larger the retinal area stimulated with strong plus lenses, the greater the effect in slowing the progression of myopia. Walline et al. 175 fit 40 children with soft multifocal contact lenses (Proclear Multifocal “D”; CooperVision, Fairport, NY) with a +2.00 D add power and compared them to a historical age-matched control group of SV distance lens wearers. The adjusted mean progression of myopia at 2 years was −1.03±0.06 D for the SV contact lens wearers and −0.51±0.06 D for the soft multifocal contact lens wearers ( P <0.0001). The adjusted mean axial elongation was 0.41±0.03 and 0.29±0.03 mm for the SV and soft multifocal contact lens wearers, respectively ( P <0.0016). Soft multifocal contact lens wear resulted in a 50% reduction in the progression of myopia and a 29% reduction in axial elongation during the 2-year treatment period. One may question Walline's findings, however, because they were compared to a historical control. In addition, it is well known from the COMET studies that the greatest myopia-controlling effect of any intervention happens in the first year. Lastly, there are no data on discontinuing the lenses with possible rebound effects.
In a recent study, children, who were found to have myopic progression after 1 year of traditional contact lens or spectacle use, were placed in 1 of 3 groups: (1) radial refractive gradient (SRRG) contact lenses, (2) OK, and (3) a SV glasses. 176 The SRRG is an experimental soft contact lens with a distance center and high plus in the mid-periphery. After 2 years, the mean myopia progression values for the SRRG, OK, and SV groups were −0.56, −0.32, and −0.98 D, respectively. This represents a reduction in myopic progression of 43% and 67% for the SRRG and OK groups as compared to the SV group. In addition, the AL increase was less by 27% and 38% in the SRRG and OK groups as compared to the SV group. Although these results are encouraging, the SRRG lens is not currently commercially available. In a different study, Aller et al. 177 used an Acuvue Bifocal (Johnson & Johnson, Jacksonville, FL) center distance bifocal soft contact lens in selected myopic esophoric patients and achieved almost a 70% reduction of myopia after 1 year, but the applicability of this finding outside of esophoric patients is not known. A meta-analysis (Fig. (Fig.6), 6 ), which included 587 subjects, from 8 studies found that concentric ring and distance centered multifocal designs slowed myopia progression by 30% to 38% and 31% to 51% for axial elongation over 24 months. 178 Turnbull et al. 179 performed a retrospective case series analysis of 110 myopic children and reported that multifocal soft lens and OK slowed myopic progression equally, that is, OK before treatment progression X=−1.17 to after treatment −0.09 D/year; dual focus soft contact lens before progression X=−1.15 to after treatment −0.10 D/year. Using a similar retrospective case series analysis as was used by Turnbull et al., 179 Cooper et al. 180 performed a retrospective case series of 32 myopic children and reported that a center distance extended depth of focus soft multifocal contact lens design slowed myopic progression with before progression X=−0.85 D to after treatment −0.04 D/year right eye and before progression X=−0.90 D to after treatment −0.04 D/year left eye.

FIG. 6. Meta-analysis of multifocal contact lenses. Meta-analysis, 178 which included eight studies published between 1999 and 2016, that compared single-vision soft lenses with both concentric ring bifocal soft contact lenses (CCML) 170 , 177 , 255 – 257 and peripheral add soft contact lenses (MCL). 171 , 175 , 176 There was less myopia progression with both lenses (the CCML had a weighted mean difference [WMD] of 0.31 D and reduced axial elongation WMD of –0.12 mm, whereas MCL had a WMD of 0.22 D and less axial elongation of 0.10 at the end of 1 year). This represented a 31% reduction of progression with the CCML and 51% reduction with MCL. Axial length reduction was also noted: 38% with the CCML and 51% with MCL after 2 years. Reprinted with permission from Li SM, Kang MT, Wu SS, et al. Studies using concentric ring bifocal and peripheral add multifocal contact lenses to slow myopia progression in school-aged children: a meta-analysis. Ophthalmic Physiol Opt 2016.
Figure Figure6 6 presents a meta-analysis of the use of soft multifocal contact lenses to slow the progression of myopia. 178
Pharmaceutical Agents
In addition to manipulating visual input with lenses to control myopia, atropine has been shown to slow the progression of the disease. Atropine was first used by Wells in 1900 to stop the progression of myopia by “paralyzing” accommodation. Analysis of a number of retrospective studies using atropine has shown that 1% atropine tends to slow the progression of myopia by almost 80% (Table (Table1 1 ). 181 – 193 The effect is by a nonaccommodative mechanism, 70 , 80 , 194 because a number of studies have shown that atropine inhibits AL: in animals that have no accommodative mechanism 195 ; when the optic nerve has been cut thus eliminating feedback necessary for accommodation 74 ; or when regionally induced AL changes occur from blur. 71 Though the exact mechanism by which atropine inhibits myopia progression is unknown, multiple studies have indicated that atropine has an effect altering the sclera. 196 – 198 It has, also, been suggested that ultraviolet (UV) light (secondary to pupil dilation) may increase collagen cross-linking within the sclera, thereby slowing scleral growth. 199
Retrospective Studies of Atropine 1% to Slow Myopic Progression

The most common complaints when using atropine, however, are pupil dilation and temporary paralysis of accommodation. These issues can be mitigated by photochromic, PAL glasses. There have been, also, some concerns of increased UV exposure and long-term retinal damage. 200 However, UV exposure (other than oblique rays) can be reduced by the use of UV coatings on the lenses, and the lost accommodation can be mitigated by the use of PALs. Besides enjoying a good safety profile with long-term clinical use, 201 Electroretinogram results (which are a sensitive indicator for early retinal damage) are normal in patients using atropine for a long term. 81 , 201 – 203 In the 2-year ATOM study (N=400), there were no serious adverse effects. Reasons for withdrawal included: allergic or hypersensitivity reactions, discomfort (4.5%), glare (1.5%), blurred near vision (1%), logistical difficulties (3.5%) and others (0.5%). Similar minimal adverse rates have been reported by other atropine studies. The use of 1% atropine seems to have its strongest effect in year one. Many of these earlier studies demonstrated long-term effectiveness of atropine 186 , 189 , 192 (Table (Table1). 1 ). Chiang et al. 204 studied the effect of 1% atropine used once a week for 1 month to 10 years. They reported a mean progression rate of 0.08 D/year in the compliant group and 0.23 D/year in the partially compliant group.
Chua et al. 205 (ATOM1) studied the effect of 1% atropine in a group of 400 children (13.5% dropout rate) where one group received atropine, whereas the other group received a placebo. Only one eye of each child was chosen for treatment. The mean progression in the control eye after 2 years was 0.6 D/year and in the atropine-treated eye was 0.14 D/year. This represents a 77% reduction in the progression of myopia. Furthermore, the AL measurements in the eyes, which received atropine, remained essentially unchanged (0.02 mm over 2 years). There were no serious adverse events with the atropine being well tolerated. Figure Figure7 7 depicts the percentage of progression in patients on 1% atropine versus control.

Atropine 1% versus control in slowing myopic progression. Data from the ATOM 1 study are pictorially presented and clearly show the effectivity of atropine over control. 205 Seventy percent of the atropine subjects had less than 0.5 D of progression compared with less than 20% of the controls. It is apparent that atropine 1% results in strong control of myopia progression. Reprinted with permission from Cooper J, Schulman E, Jamal N. Current status on the development and treatment of myopia. Optometry 2012;83:179–99.
There have been a number of studies that evaluated the relationship of dosage of atropine to the reduction of progression. Shih et al. 206 evaluated the effect of different doses of atropine on 200 children (6–13 years of age) who were randomly prescribed one drop of 0.5%, 0.25%, or 0.1% atropine, or 0.5% tropicamide (control group) in both eyes nightly. The mean progression of myopia was 0.04±0.63 D/year in the 0.5% atropine group, 0.45±0.55 D/year in the 0.25% atropine group, and 0.47±0.91 D/year in the 0.1% atropine group, as compared to 1.06±0.61 D/year in the control group. At the end of the 2-year treatment period, 61% of children in the 0.5% atropine group, 49% in the 0.25% atropine group, and 42% in the 0.1% atropine group had no myopic progression (Fig. (Fig.8). 8 ). In another study, the concentration of atropine was varied from winter (0.5%) to summer (0.1%) based on the assumption that myopia progresses less during the summer. This allowed children to have less pupillary dilation during the summer months when the sunlight and photophobia is the greatest. 207 This regimen slowed myopic progression by 77%. Fang et al. 208 evaluated the efficacy of 0.025% atropine to prevent the development of myopia in a group of children presenting with the signs of myopic progression. There was a 50% reduction in the number of children who converted from emmetropia to myopia. The highest concentration of atropine, which does not cause any symptoms related to pupil dilation or decreased accommodation when atropine is used is 0.02%. 209

Myopic progression with various doses of atropine. Shih et al. demonstrated that the ability of atropine to control progression is directly related to concentration. 206 The higher the dosage, the more effective atropine is in slowing the progression of myopia. It is clear that even at a relatively low dosage of atropine 0.01%, there is a clinically effective retardation of the progression of myopia. Reprinted with permission from Shih YF, Chen CH, Chou AC, Ho TC, Lin LL, Hung PT. Effects of different concentrations of atropine on controlling myopia in myopic children. J Ocul Pharmacol Ther 1999;15:85–90.
The ATOM 2 study 200 evaluated various concentrations of atropine, including the one below that threshold, that is, 0.5%, 0.1%, and 0.01%. After 2 years, researchers found that all 3 concentrations slowed the progression of myopia. The mean progression with each concentration (spherical equivalent) was 0.15 D/year (0.5% atropine), 0.19 D/year (0.1% atropine), and 0.25 D/year (0.01% atropine) 210 (Fig. (Fig.9). 9 ). On first glance, the ATOM 2 study suggests that myopic progression was slowed with all concentrations, with similar effects between moderate and low concentrations. However, this conclusion is not justified if one uses AL to measure myopic progression rather than refractive error. Figure Figure10 10 depicts the axial change measurements from ATOM 1 and 2 studies combined at the end of 2 years. The ATOM 1 study showed a minimal 0.02 mm change in AL over 2 years of time with the use atropine 1%, whereas the ATOM 2 showed no statistical difference between the placebo and atropine 0.01% group. This is important for two reasons. First, if the primary purpose of slowing myopia progression is to reduce axial elongation which in turn decreases future retinal complications, then the lower concentrations are not nearly as effective as atropine 1%. Second, minimal difference between placebo and atropine 0.01% AL changes versus significant refractive changes between placebo and atropine 0.01% should make the clinician question of the “true effect” of atropine 0.01%.

Progression of myopia during 3 phases of ATOM studies. This graph depicts the cycloplegic refractions (spherical equivalent) in all 3 phases of the ATOM 1 and 2 studies. 212 The first phase was for 2 years during which subjects were randomized to receive various concentrations of atropine (1%, 0.5%). After 2 years, treatment was stopped in all groups for 1 year of time. Those patients still showing more than 0.50 diopters of myopia progression were placed on atropine 0.01% and followed for another 2 years. Reprinted with permission from Chia A, Lu QS, Tan D. Five-year clinical trial on atropine for the treatment of myopia 2: Myopia control with atropine 0.01% eyedrops. Ophthalmology 2016;123:391–99.

Changes in AL and SPH EQ after 2 years of treatment. Figure 10 depicts the changes in axial length in millimeters (yellow bars going up); spherical equivalent in diopters calculated from the axial length data (red going down); and cycloplegic automated refractor measurements in diopters (green going down) at the end of the 24-month treatment period. The measurements were derived from the ATOM 1 study for atropine 1% and placebo and ATOM 2 for atropine 0.01%, 0.1%, and 0.5%, respectively. It is readily apparent that there is no real difference between axial length measurements after 24 months between placebo and atropine 0.01%; moderate changes with atropine 0.1% and 0.5%; and dramatic changes with atropine 1% (yellow bars). However, the spherical equivalent measurements (green bars), compared with placebo in diopters, show a much greater change over time again being greatest for atropine 1%. The difference between the effect of atropine 0.01% and atropine 1% is not nearly as great as the concentration differences.
After 2 years, all participants in the ATOM 2 study discontinued the use of atropine for 1 year. At the end of that year, 24% of the 0.01% group, 59% of the 0.1% group, and 68% of the 0.5% groups in the original ATOM 2 trial progressed more than 0.5 D of myopia and were retreated with 0.01% atropine for an additional 2 years. 211 This rebound effect was much greater with cycloplegic refractions as compared to AL changes. The “rebound effect” observed in the ATOM studies can be partially explained by the fact that atropine has greater cycloplegic effect than 1% cyclopentolate used for the follow-up refractions, creating an impression that atropine slows the progression of myopia more than it really does in the first year. This creates an impression of the rebound effect, which is, in fact, much smaller than what is observed when doing cycloplegic refractions. Because atropine suppresses the signal for axial elongation, an abrupt stopping of higher dosages would result in faster elongation than discontinuation of lower concentrations. These findings suggest that atropine use should be tapered down rather than be abruptly discontinued. 212
After stopping the use of atropine drops for 1 year, the patients were re-assessed. 213 It was found that the progression of myopia have resumed in some patients or appeared to have completely stopped in others. Those patients, in whom progression stopped, were presumed to be abated (future data are needed to substantiate this claim), whereas progression continued in others. Those with continued progression after phase 2 were restarted on 0.01% atropine and reassessed 2 years later (total of 5 years). 211 Those who did not progress after the discontinuation phase usually did not progress during the next 2 years of observation. The authors concluded that 0.01% atropine was more effective than the higher dosages in slowing the progression of myopia. A recent meta-analysis suggests that there is no clinical difference between the effectively of low and high concentrations of atropine to slow the progression of myopia. 214 As mentioned previously, this conclusion must be viewed cautiously in light of AL measurements.
The rebound results also need to be evaluated with caution: clinicians do not usually put patients on 1% atropine for 2 years and then stop the medication. Patients are usually treated with atropine for many years without interruptions. Studies, in which 1% atropine has been used for many years, found that atropine did not lose its effectiveness over the long run. The subjects who were least affected by the atropine treatment had the following characteristics: (1) 2 myopic parents, (2) developed myopia earlier, and (3) progressed more than the average of 0.66 D/year.
The 5 years of data suggest that 0.01% atropine was more effective (and with fewer side effects) in slowing progression of myopia compared with higher concentrations of the drug. One must keep in mind, however, that in normal clinical practice, atropine treatment is typically continued for more than 2 years without interruption. In summary, these findings suggest that myopia did not progress with 0.01% atropine in the first 2 years of the study. Myopia did not progress once treatment was stopped (discontinuation phase), and individuals no longer needed further treatment to slow myopia.
Those who progressed more than 0.5 D, when atropine was discontinued, were more likely to have been on a higher dosage and needed further treatment. These data suggest that over the long run, 0.01% atropine is more effective than higher concentrations and causes minimal symptoms secondary to pupillary dilation or loss of accommodation, and the 0.01% concentration can be used for 5 years and then stopped. If progression recurs, treatment with 0.01% atropine can be resumed. If higher concentrations of atropine are required atropine 0.02% may be tried, the treatment should be stopped gradually by tapering down the concentration of the drug. 209 The ATOM 2 study does provide compelling support to begin treatment of myopia with 0.01% atropine, but our clinical experience is that it might be less effective than suggested. In addition, if one uses AL measurements rather than refractive error to monitor effectively of 0.01% atropine versus control to slow myopic progression, it is apparent that there is no difference between the 2 treatment arms.
Time Spent Outdoors
Several recent studies suggest that time spent outdoors slows both onset and progression of myopia in children. 215 – 224 It was also found that the effect of outdoor activities on myopia is not necessarily related to physical activity and that the shear exposure to outdoor environment has therapeutic effect. 217 These findings triggered a number of investigations trying to pinpoint the exact factor(s) responsible for the effect of outdoor activities on myopia. Several studies suggested that exposure to brighter light, increased levels of vitamin D, increased levels of dopamine, or UV light by itself are responsible for the effect of outdoors on myopia onset and progression. 225 – 234 However, further studies essentially ruled out the role of vitamin D and UV light in the inhibition of myopia development by exposure to outdoors. 235 , 236
Recently Torii et al. 234 demonstrated that violet light (VL) (360–400 nm wavelength) suppresses myopia progression in chicks and humans. They retrospectively measured the AL elongation among myopic children, who wore either VL blocked eyeglasses or one of two types of contact lenses (partially VL blocking and VL transmitting). They found that the VL transmitting contact lenses suppressed myopia progression more than VL blocking lenses. They suggested that because VL exposure is limited by UV protection from being indoors; filtered out UV by panel window glass; and filtered out UV by glasses, some contact lenses and sunglasses that increased VL exposure may be a preventive strategy against myopia progression.
There is, also, evidence that increasing the illumination in classrooms decreases the incidence of myopia. 237 Bright light was also shown to inhibit form-deprivation myopia and reduce lens-induced (defocus-induced) myopia in animal studies. 227 – 231 However, there is no information whether bright light might have caused animals to close their eyes because of photophobia caused by high light intensity, thus, reducing visual input. Moreover, studies that assessed the effect of outdoors on myopia did not take into account the use of sunglasses in bright light, which would reduce the importance of bright light exposure and emphasize other factors. Such factors would be the overall substantial differences in the visual environment between indoors and outdoors. 238 The indoor activities create far more hyperopic defocus (causing myopia) across the entire surface of the retina than any outdoors activities. Outdoor activities essentially eliminate any defocus across the entire visual field that serves as a stop signal for the eye growth (thus, inhibiting development of myopia). Brighter light intensity also leads to pupil constriction and increased depth of focus, which reduces optical blur and increases contrast. Change in contrast, in turn, would affect the function of amacrine cells, which might explain the role of dopamine in myopia development in animal models. Although the exact mechanism responsible for the effect of outdoor activities on myopia is unknown, spending more time outdoors clearly has a substantial therapeutic effect on myopia onset and possibly progression. Therefore, it should be recommended that children, especially those who have two myopic parents or show signs of myopia development or progression, spend more time outdoors as preventive measure of developing myopia.
CONCLUSIONS
In summary, there is strong evidence that myopia is a result of an interaction between genes and environment and can be slowed by a variety of treatments. Parents should be aware of what is and is not effective including the risks and benefits associated with each treatment option (Figs (Figs11 11 and and12). 12 ). Despite none of these interventions having FDA approval/clearance at this time to treat myopia progression, we believe that with informed consent, an appropriate treatment plan should be instituted. Today, treatment preferences seem to vary by country and profession. More eye care professionals in China advocate the use of OK; whereas in Taiwan and Singapore, more advocate atropine; and in the United States, some eye care professionals prescribe soft multifocal contact lenses, and/or advocate OK and some ophthalmologists advocate atropine. In Taiwan, over 60% of the children with myopia are on atropine. 239 Recently, there are data supporting the additive effects of optically correcting myopic children with OK and low dosages of atropine, that is, after a year in the study “OK only patients” increased AL by 0.19 mm, whereas “OK and atropine” increased AL by 0.09 mm. 240 Because they use different stop mechanisms, it is not surprising that their effects are additive. There is obviously the need for more studies into the mechanisms of myopia and refractive eye development, but the future is encouraging.

Meta-analysis of 16 different treatments. A meta-analysis 243 of 16 different treatments for myopia was performed using a comparison with either placebo or single-vision spectacle lenses with the following: high-dose atropine (refraction change: 0.68; axial length change –0.21); moderate-dose atropine (refraction change: 0.53; axial length change: –0.21); low-dose atropine (refraction change: 0.53 axial length change: –0.15); pirenzepine (refraction change: 0.29; axial length change: –0.09); OK (axial length change: –0.15); multifocal contact lenses (axial length change: –0.11); and progressive-addition spectacle lenses (refraction change: 0.14 axial length change). Reprinted with permission from Huang J, Wen D, Wang Q, et al. Efficacy comparison of 16 interventions for myopia control in children: A network meta-analysis. Ophthalmology 2016;123:697–708.

Percentage of reduction of myopia progression with various treatments. Calculated percentage of reduction of progression of myopia for each treatment. Meta-analysis numbers 243 were used to calculate the reduction in progression. Meta-analysis only included prospective clinical trials. Cooper et al. 81 previously calculated the reduction in progression from all published studies without regard to methodology.
Ongoing research already provided some insights into molecular pathways underlying myopia and could be expected that it will soon produce new drug targets and drugs for treatment of myopia. In the meantime, children who have high-risk factors (myopia first noted around 4 or 5 years. with aggressive progression and parental myopia) should probably be started with 1% atropine. One might consider the prophylactic use of atropine 0.01% in children with a strong risk of development of myopia, that is, 2 parents being myopic and decreasing hyperopia of 0.5 D/year. On the other hand, children who become myopic after the age of 8 can be treated with 0.01% atropine, soft multifocal contact lenses or OK. In addition, patients with more than 6 diopters of myopia can wear soft multifocal contact lenses or a combination of OK contact lenses and glasses to obtain an effective treatment result. 241 Because soft multifocal contact lenses, OK and low dosage of atropine seem to be equally effective, 242 patient concerns and compliance may help guide treatment selection.
Some children tend to prefer their glasses and thus require atropine. Children who are more athletic usually prefer OK or soft multifocal contact lenses. Parents who are fearful of overnight contact lens wear often choose low concentrations of atropine or soft multifocal contact lenses, whereas parents/patients concerned about the long-term effects of atropine usually choose OK. Some patients are concerned with the risks of OK associated with sleeping in lenses, whereas others are concerned about the long-term effects of atropine even at low dosages. In addition, distance center soft contact lenses may have a better indication in myopes with less than −2.00 D because effectiveness is related initial refractive error. We often prescribe distance center soft multifocal contact lenses even though the published clinical evidence is not yet as strong; however, the perceived risk is less. Finally, children should be encouraged to spend more time outside and the public and policymakers should be informed of the potential benefits of outdoor activities, so that school schedules, perhaps, could be adjusted to allow more time for outdoor activities during school hours and after school.
J. Cooper: Consultant to VTI (Visioneering Technologies, Inc., Alpharetta, GA), Treehouse Eyes, and Magic Leap, the remaining author has no funding or conflicts of interest to disclose.
Understanding Myopia: Pathogenesis and Mechanisms
- Open Access
- First Online: 10 October 2019
Cite this chapter
You have full access to this open access chapter
- Ranjay Chakraborty 3 ,
- Scott A. Read 4 &
- Stephen J. Vincent 4
35k Accesses
11 Citations
91 Altmetric
Myopia is a common refractive error, characterized by an excessive increase in axial length relative to the refractive power of the eye. Despite much research, the mechanisms underlying the development of myopia are unknown. A large body of work on animal models (such as chicks, guinea pigs, and monkeys) has been instrumental to our understanding of visually guided ocular growth, and potential mechanisms leading to myopia. These studies have shown that experimentally degrading the quality of the image formed on the retina by introducing translucent diffusers (i.e., form-deprivation), or altering the focal point of the image with respect to the retinal plane by imposing plus or minus lenses to the eyes (i.e., lens induced defocus) results in abnormal eye growth and development of reflective errors. Ocular changes in response to form-deprivation and lens induced defocus are primarily associated with changes in axial length (mainly due to changes in vitreous chamber depth) and choroidal thickness. These experimentally induced ocular changes quickly revert to normal upon removal of the imposed optical treatment. Physiological changes in retinal cells and neurotransmitters (such as dopamine), presence of ocular aberrations, altered accommodative response to visual stimuli, and even subtle variations in natural circadian rhythms of axial length may all influence ocular growth, and hence susceptibility to myopia. In fact, several optical interventions alter ocular aberrations, peripheral refraction, and the accommodative response of the eye in an attempt to arrest myopia development. Epidemiological studies have also linked excessive near work, better socioeconomic status, and urbanization to myopia, although the exact cause for these associations remain elusive. Based on decades of work on the effects of ambient lighting on refractive development in laboratory animals, recent clinical studies have revealed protective effects of greater outdoor exposures on development and progression of myopia in children. Experimental models continue to provide valuable information on the cellular and biochemical mechanisms of myopia.
You have full access to this open access chapter, Download chapter PDF
Similar content being viewed by others
Animal Models of Experimental Myopia: Limitations and Synergies with Studies on Human Myopia
The RPE in Myopia Development
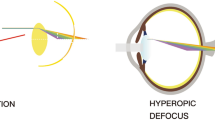
Light Signaling and Myopia Development: A Review
- Emmetropization
- Axial length
- Form-deprivation
- Accommodation
Visual environment (or the quality of the retinal image) modulates the refractive development of the eye.
Ocular response to form-deprivation and lens induced defocus is evident across a wide range of animal species, including humans.
The visual system appears to be more sensitive to myopic than hyperopic defocus.
Evidence suggests that greater time spent outdoors is protective against development and progression of myopia in children.
4.1 Emmetropization and Normal Ocular Growth in Human Eyes
When incident parallel rays of light from distant objects are brought to a focus upon the retina without accommodation, it is known as emmetropia. During postnatal eye growth, the precise matching of the axial length (the distance from the anterior corneal surface to the retina along the visual axis) and the optical power of the eye brings the eye to emmetropia [ 1 , 2 ]. This active regulatory process that harmonizes the expansion of the eye with the optical power of the cornea and the crystalline lens is known as emmetropization [ 1 ]. Any disruption to these highly coordinated ocular changes results in the development of refractive errors, wherein distant images are focused either behind (hyperopia) or in front (myopia) of the retina [ 1 ].
Human eyes exhibit a distinctive pattern of eye growth during the early period of visual development. The distribution of refractive errors at birth appears to be normally distributed [ 3 , 4 ]. Apart from some exceptions [ 5 ], the majority of newborn infants are moderately hyperopic (~+2.00 to +4.00 D) and this refractive error reduces significantly during the first 18 months of life [ 3 , 6 , 7 , 8 ] (Fig. 4.1 ). By about 2–5 years of age, the distribution becomes leptokurtic with a peak around emmetropia to low hyperopia of about +0.50 to +1.00 D [ 5 , 6 , 8 , 11 , 12 ]. Although studies have reported small reductions in hyperopic refraction until the middle to late teen years [ 13 , 14 ], emmetropization is believed to be largely completed by 5–6 years of age [ 5 , 6 , 8 , 14 ].
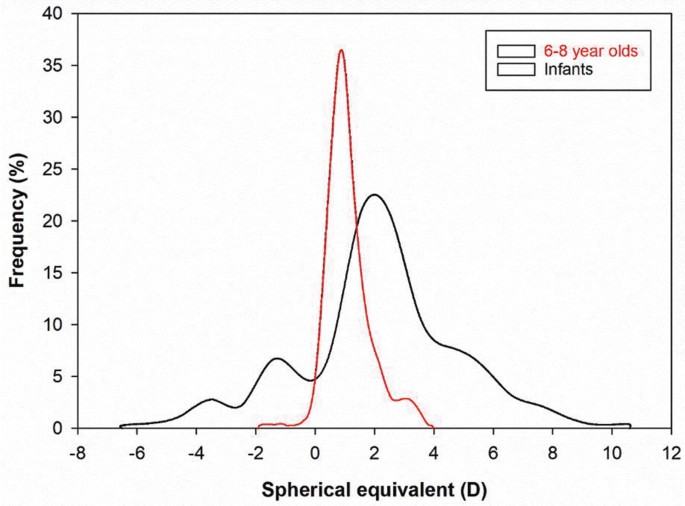
Comparison of refractive error distribution among newborns [ 3 ] and 6–8-year-old children [ 9 ]. The distribution of refractive errors narrows between infancy to early childhood during the process of emmetropization. Adapted from FitzGerald and Duckman [ 10 ]
Based on the visually guided ocular growth observed in a variety of animal models [ 15 , 16 , 17 ] (see Sect. 4.3 ), the growth of the human eye is also believed to be modulated by an active visual feedback from the hyperopic refractive error in neonatal eyes [ 18 ]. Studies have found a strong correlation between the rapid reduction in hyperopia and the changes in axial length during early ocular development [ 18 , 19 ]. Human eyes are ~17 mm long after birth and grow to about 20 mm after the first year [ 11 , 18 , 19 , 20 ]. This rapid expansion of the eye is largely attributed to the expansion of the vitreous chamber [ 18 , 21 ]. From 2–3 years of age, axial elongation slows to approximately 0.4 mm/year until preschool age [ 11 ]. Consistent with changes in ocular refraction, the growth of the eye stabilizes further at 5–6 years, and only increases by 1–1.5 mm through the teenage years [ 11 , 20 , 21 ]. Together, these studies suggest that axial length is the most influential factor for emmetropization in human eyes.
In addition to changes in axial length, there is also a significant reduction in the refractive power of the cornea and the crystalline lens that contributes to the overall reduction in hyperopia in the first year of life [ 11 , 18 , 22 ]. Mutti et al. [ 18 ] reported a reduction of 1.07 and 3.62 D in corneal and crystalline lens powers, respectively, associated with flattening of the corneal and lens radii in newborn infants, between the ages of 3 and 9 months. Studies have also found higher degrees of corneal astigmatism in newborn infants [ 23 , 24 , 25 , 26 , 27 , 28 ], which reduces during the first 4 years of life and is associated with corneal flattening [ 24 , 27 , 29 , 30 ]. Overall, these studies suggest that emmetropization in human eyes is largely attributed to the changes in axial length with minor contributions from corneal and crystalline lens powers.
Refractive errors occur as a result of either variations in (a) axial length with respect to the total refractive power of the eye (termed axial myopia or hyperopia) or (b) refractive power of the cornea and the crystalline lens with respect to the axial length of the eye (termed refractive myopia or hyperopia). This chapter focuses on the pathogenesis and potential underlying mechanisms of myopia, and the following section discusses the changes in different ocular parameters during myopic eye growth.
4.2 Ocular Biometric Changes in Human Myopia
As discussed earlier, the axial length of the eye is the primary biometric determinant of refractive error; however, the dimensions, curvature, and refractive index of each individual ocular structure contribute to the final refractive state. Ocular biometrics vary considerably throughout childhood, during the development and progression of myopia, and in response to clinical myopia control interventions.
4.2.1 Cornea
Several cross-sectional analyses have revealed a weak association between increasing corneal power (a steeper radius of curvature) and increasing levels of myopia [ 31 , 32 , 33 ], while others report no association [ 34 ], or the opposite relationship [ 35 ]. Longitudinal studies indicate that changes in corneal curvature during childhood [ 36 , 37 , 38 ] and early adulthood [ 39 ] are minimal and not associated with the magnitude of myopia progression. However, since the correlation between spherical equivalent refraction (SER) and the axial length to corneal radius ratio is typically stronger than that of axial length alone (by 15–20%) [ 40 , 41 , 42 , 43 ], corneal curvature does appear to make a modest contribution to the magnitude of myopia. Although corneal thickness does not vary systematically with refractive error [ 44 , 45 , 46 , 47 ], a reduction in corneal hysteresis (an estimate of corneal biomechanical strength or viscoelasticity) has been observed with increasing levels of myopia in children [ 48 , 49 ] and adults [ 50 , 51 , 52 ]. However, the causal nature of this relationship remains unclear, or if such corneal metrics correlate with posterior scleral biomechanics.
4.2.2 Crystalline Lens and Anterior Chamber Depth
While the cornea flattens substantially during infancy and then remains relatively stable throughout childhood, the crystalline lens continues to thin, flatten, and reduce in optical power until approximately 10 years (a 0.25–0.50 D reduction per year), concurrent with lens fiber compaction [ 53 , 54 , 55 ]. These changes may be part of an emmetropization mechanism to compensate for continued axial elongation or a mechanical consequence of equatorial eye growth. Across a range of ethnicities, Mutti et al. [ 56 ] observed that within 1 year of myopia onset, compensatory crystalline lens thinning and flattening abruptly halted compared to children who remained emmetropic (Fig. 4.2 ), suggesting that childhood myopia is not purely axial in nature, but involves a decoupling of highly correlated anterior and posterior segment eye growth. In Singaporean children, Iribarren et al. [ 57 ] reported a transient acceleration in the reduction of lens power during myopia onset when the rate of axial growth was high, which was not sustained as myopia progressed (Fig. 4.3 ). Paradoxically, after 10 years of age, the lens continues to thicken and increase in curvature with the bedding down of additional fibers, but reduces in optical power, most likely due to a steepening of the gradient refractive index [ 58 ]. Changes in anterior chamber depth throughout childhood are inversely related to changes in lens thickness (as the lens thins, the anterior chamber deepens), and the anterior chamber is typically deeper in myopes compared to emmetropes and vice versa for the crystalline lens [ 53 ].
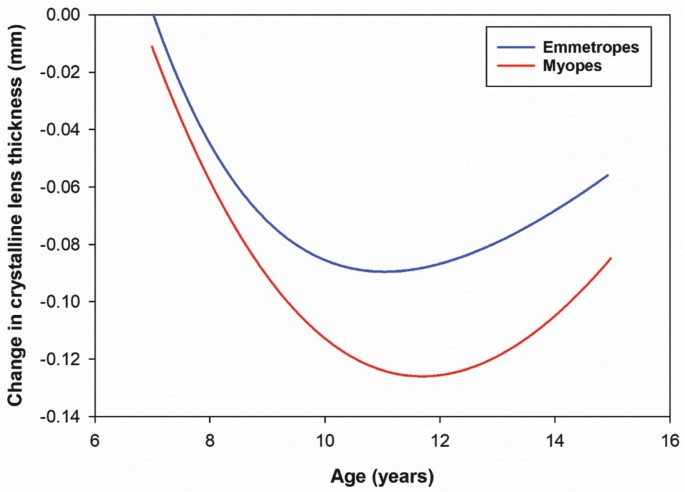
The change in crystalline lens thickness as a function of age in myopes and emmetropes during childhood (after Mutti et al. [ 56 ]). Shortly after myopia onset (10–12 years), compensatory crystalline lens thinning abruptly halted compared to children who remained emmetropic suggesting that myopia development involves a decoupling of anterior and posterior segment eye growth
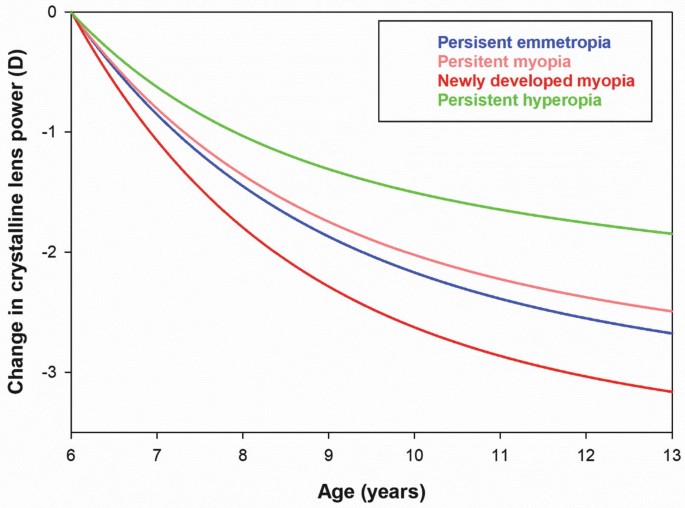
The change in crystalline lens power during childhood (calculated from refraction and biometric measurements) in young Singaporean children for a range of refractive error groups (after Iribarren et al. [ 57 ]) The reduction in lens power increases during myopia onset (to a greater extent than other refractive error groups), but is not sustained throughout myopia progression
4.2.3 Vitreous Chamber and Axial Length
In contrast to the anterior segment, changes in the posterior segment (particularly, the vitreous chamber, choroid, and sclera) are more pronounced in myopic compared to non-myopic eyes (Fig. 4.4 ). Axial length, or more precisely, the vitreous chamber depth is the primary individual biometric contributor to refractive error in children, young adults, and the elderly [ 34 , 59 , 60 ], with the vitreous chamber depth accounting for over 50% of the observed variation in SER, followed by the cornea (~15%) and crystalline lens (~1%) [ 60 ]. Modeling of cross-sectional and longitudinal data from emmetropic children indicates that axial length and vitreous chamber depth increase by approximately 0.16 mm per year from age 6–10 years, slowing to 0.05 mm per year from 11 to 14 years [ 61 ]. In myopic children aged between 6 and 11 years (corrected with single vision spectacles or contact lenses) average growth rates of approximately 0.30 mm per year have been reported [ 37 , 62 , 63 ], with greater vitreous chamber and axial elongation observed in younger females with myopic parents [ 37 ]. A range of myopia control interventions significantly slow the rate of eye growth and myopia progression during childhood, in some cases by up to 50% [ 64 ], and this reduction in axial elongation appears to be initially modulated by changes in the choroid underlying the retina.
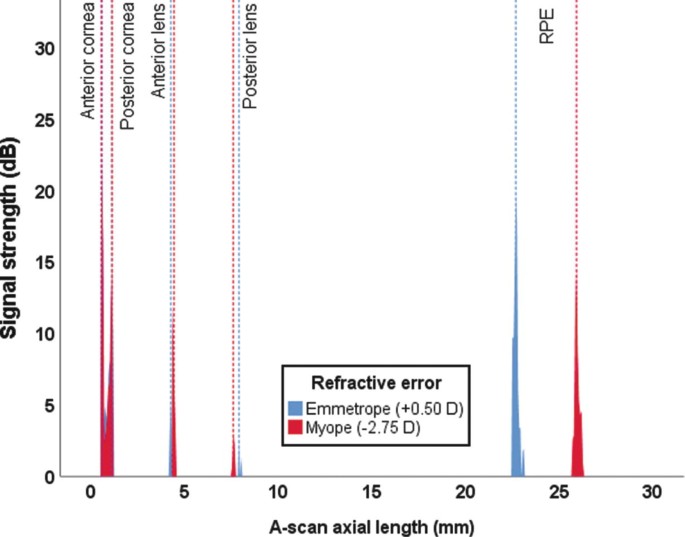
Optical low coherence reflectometry A-Scan output from two 11-year-old males (one myope and one emmetrope). The predominant biometric differences are the deeper vitreous chamber (19.05 mm compared to 15.47 mm) and the longer axial length (25.87 mm compared to 22.69 mm) in the myopic eye. The anterior chamber depth is slightly shallower in the emmetropic eye (by 0.13 mm) while the crystalline lens is thicker (by 0.49 mm) in comparison to the myopic eye. The corneal thickness varies by only 0.04 mm
4.2.4 Choroid
The choroid supplies the outer retina with oxygen and nutrients and regulates intraocular pressure and ocular temperature. The choroid is typically thinner in myopic compared to non-myopic eyes (most pronounced at the fovea [ 65 , 66 ]) and thins with increasing myopia and axial length in both adults [ 67 , 68 , 69 , 70 , 71 , 72 , 73 , 74 ] and children [ 75 , 76 , 77 ]. Significant choroidal thinning is also observed in high myopia (<−6.00 D) or eyes with posterior staphyloma [ 78 ], and has been associated with the presence of lacquer cracks [ 79 ], choroidal neovascularization [ 80 ], and reduced visual acuity [ 81 ]. The choroid also appears to be a biomarker of ocular processes regulating eye growth given that the central macular choroid thins during the initial development and progression of myopia [ 82 , 83 , 84 ] and thickens in response to imposed peripheral myopic retinal image defocus [ 85 , 86 ], topical anti-muscarinic agents [ 87 , 88 ], and increased light exposure [ 89 ] (clinical interventions associated with a slowing of eye growth in children).
4.2.5 Sclera
Scleral thinning associated with axial myopia is primarily restricted to the posterior pole [ 90 , 91 , 92 ], due to scleral tissue redistribution [ 93 ]. Scleral thinning may alter the tissue strength surrounding the optic nerve head, rendering myopic eyes more susceptible to glaucomatous damage [ 94 , 95 ]. Consequently, posterior reinforcement surgery using donor scleral tissue has been refined over the years to arrest further axial elongation and scleral thinning in highly myopic eyes [ 96 , 97 ]. Although anterior scleral thickness is similar between myopic and non-myopic eyes [ 98 , 99 , 100 ], there is growing evidence that the anterior sclera thins slightly during accommodation, particularly in myopic eyes [ 101 , 102 ], most likely due to biomechanical forces of the ciliary muscle. A greater thinning observed in myopic eyes may be a result of a thicker posterior ciliary muscle [ 103 , 104 , 105 ] or changes in biomechanical properties of the sclera reported in animal models of form-deprivation myopia [ 106 , 107 ].
4.3 Visual Environment, Emmetropization, and Myopia: Evidence from Animal Models
Over the last four decades, numerous animal models have provided valuable insight into the mechanisms underlying emmetropization and refractive error development. Much of the knowledge on vision-dependent changes in ocular growth has emanated from animal experiments in which either the quality of image formed on the retina is degraded (known as form-deprivation [FD]), or the focal point of the image is altered with respect to the retinal plane (known as lens induced defocus). Both FD and lens induced defocus result in abnormal eye growth and development of refractive errors. This section summarizes the attributes of experimental ametropias derived from these two visual manipulations, their differences, and significance for understanding refractive error development in humans.
4.3.1 Form-Deprivation Myopia
FD is the most commonly used experimental paradigm to model axial myopia in animals. Depriving the retina of form or patterned vision through eyelid suture [ 108 , 109 , 110 ], or translucent diffusers [ 2 , 15 , 111 , 112 , 113 , 114 ] consistently produces axial myopia (Fig. 4.5 ). The use of noncontact translucent diffusers offers a more reliable representation of ocular changes with FD since they do not induce corneal changes (unlike eyelid fusion techniques). The ocular changes observed in response to FD clearly illustrate that degrading retinal image quality can produce robust myopic changes. Schaeffel et al. [ 115 ] proposed that FD is an open-loop condition, in which myopia develops as a result of uncoordinated ocular growth due to reduced retinal image contrast (or mid-range spatial frequency vision) [ 116 ] and the absence of visual feedback related to the effective refractive state of the eye [ 117 ].
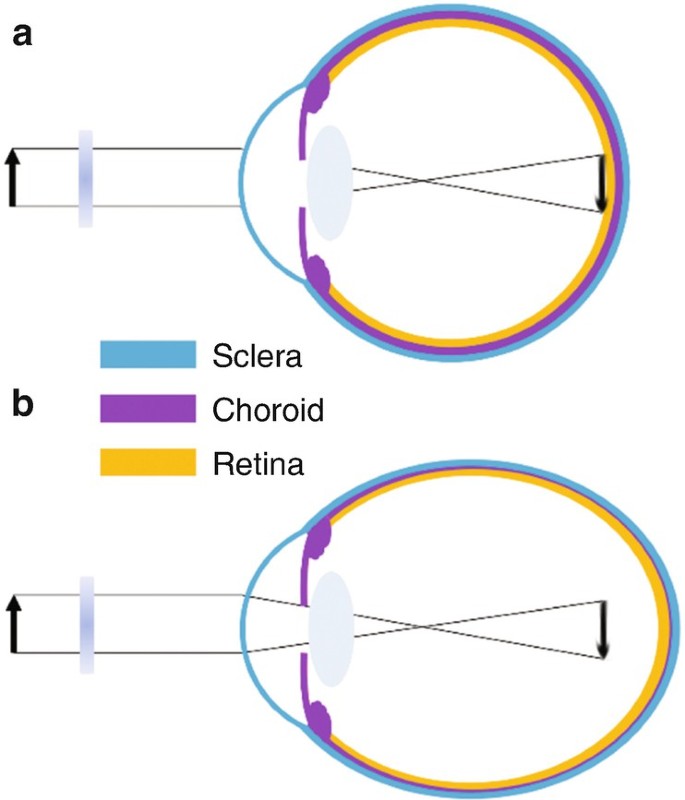
Ocular compensation for form-deprivation (FD). ( a ) A diffuser causes nondirectional blur and a reduction in contrast of the retinal image. ( b ) The absence of visual feedback related to the effective refractive state of the eye causes a thinning of the posterior choroid and an increase in ocular growth, resulting in myopia
The myopic response to FD varies among different animals. It is generally greatest in chickens (−9 D after 5 days of FD) [ 118 ], followed by tree shrews (−8 D after 12 days in young animals) [ 119 ] and guinea pigs (−6.6 D after 11 days) [ 113 ], and is less pronounced and much more variable in marmosets (−8 D after 4.5 weeks) [ 120 ] and rhesus monkeys (~−5 to −6 D after 17 weeks) [ 121 , 122 ]. Variations observed between individual studies and animal models may be due to differences in experimental paradigms, the duration and extent of FD, inherent ocular anatomical variations, and/or differences in susceptibility to environmental myopia. Nevertheless, the myopic response to FD is conserved across a wide range of animal species (including fish, rabbit, mouse, and kestrel) [ 123 ]. In all species, axial myopia is predominantly caused by a significant elongation of the vitreous chamber, along with thinning of the choroid and the sclera [ 16 , 17 , 113 , 124 , 125 , 126 , 127 , 128 , 129 , 130 , 131 , 132 ]. Few studies have also reported changes in corneal curvature and lens thickness with FD [ 131 , 133 , 134 , 135 ]. Interestingly, ocular conditions that cause varying degrees of visual deprivation in humans such as ptosis [ 136 ], congenital cataract [ 137 ], corneal opacity [ 138 ], and vitreous hemorrhage [ 139 ] are associated with myopia, which may result from mechanisms similar to FD myopia observed in animals.
FD myopia is a graded phenomenon, where increasing degrees of image degradation are positively correlated with the magnitude of induced axial myopia [ 129 , 140 ]. In addition, the effects of FD declines with age. Younger chicks [ 141 , 142 ], macaques [ 143 ], tree shrews [ 119 ], and marmosets [ 144 ] show greater ocular changes in response to image degradation compared to older animals, potentially due to age-related reductions in sensory processing of blur stimuli or changes in scleral growth [ 144 ].
4.3.2 Lens Defocus Ametropias
Perhaps the strongest evidence of visual regulation of ocular growth comes from animal studies that show eyes can actively compensate for artificially induced myopic and hyperopic defocus by adjusting the axial length to the altered focal plane (i.e., emmetropization through the treatment lenses) (Fig. 4.6 ) [ 145 ]. Myopic defocus with plus lenses simulates artificial myopia that leads to a thickening of the choroid (moving the retina forward) and a reduction in the overall growth of the eye, thus, causing a hyperopic refractive error. Conversely, hyperopic defocus with minus lenses induces artificial hyperopia that leads to a thinning of the choroid (moving the retina backward) and an increase in ocular growth, resulting in myopia to re-establish the optimal refractive state. This phenomenon was first documented in chicks [ 145 ], and has been extensively studied in chicks [ 16 , 17 , 146 , 147 , 148 ], tree shrews [ 149 ], rhesus monkeys [ 15 , 150 ], marmosets [ 151 ], guinea pigs [ 152 , 153 ], and mice [ 154 ] thereafter, indicating that the mechanisms regulating ocular growth can distinguish the sign of the imposed defocus in a wide range of animal species.
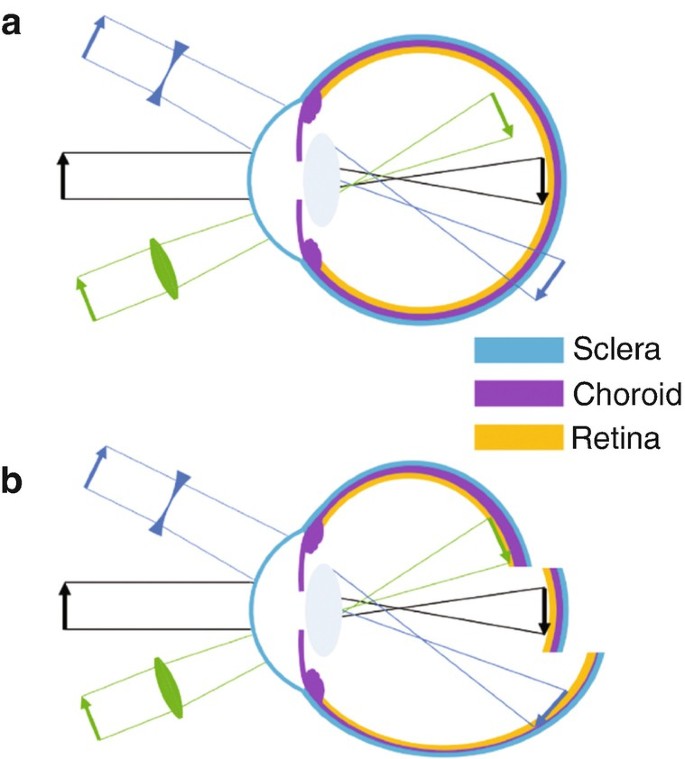
( a ) Schematic of imposed lens defocus. With no lens (black arrow), incident parallel rays of light from distant objects are focused on the retina. A plus lens (green, convex) causes the retinal image to focus in front of the retina known as myopic defocus, whereas a minus lens (blue, concave) focuses the image behind the retina known as hyperopic defocus. ( b ) A normal eye with no imposed lens defocus (black) exhibits normal ocular growth and choroidal thickness. Myopic defocus with plus lenses (green) causes a thickening of the choroid (moving the retina forward) and a reduction in the overall growth of the eye, causing a hyperopic refractive error. Hyperopic defocus with minus lenses (blue) leads to a thinning of the choroid (moving the retina backward) and an increase in ocular growth, resulting in myopia. Adapted from Wallman J and Winawer J, 2004 [ 1 ]
Chick eyes can compensate for a remarkable range of +15 to −10 D of imposed defocus [ 16 , 147 ]. However, the operating range of defocus is much smaller for other animal species (monkey: −2 to +8 D [ 15 ], marmosets: −8 to <+4 D [ 151 ], tree shrew: −10 to +4 D [ 155 ], guinea pig: −7 to +4 D) [ 152 , 156 ]. Compared to birds, the inability of primates to compensate for greater magnitudes of defocus may be due to bigger eye size [ 15 , 150 ], the process of emmetropization [ 150 ], differences in the accommodative response to defocus stimuli, and/or the degree of independent accommodation between fellow eyes [ 15 , 150 , 157 ]. In all animals, the axial response to lens induced defocus is dependent upon the power of the treatment lens [ 147 , 149 , 150 , 151 , 152 , 158 ], and is predominantly attributed to the changes in vitreous chamber depth [ 16 , 147 , 159 ]. Similar to FD, the ocular response to lens induced defocus decreases with age [ 16 ]. Recent evidence suggests that the human visual system may also be able to detect the sign and magnitude of imposed defocus and make compensatory changes in axial length, similar to other animals. A number of studies have reported small bidirectional changes in axial length and choroidal thickness in response to 1–2 h of myopic and hyperopic defocus in children and young adults [ 160 , 161 , 162 , 163 , 164 , 165 ].
Studies have shown that the biological mechanisms underlying alterations in ocular growth to myopic and hyperopic defocus may be completely different (and not merely opposite to each other), and that the visual system is perhaps more sensitive to myopic defocus [ 166 , 167 ]. In fact, the ocular response to lens induced defocus depends on the frequency and duration of lens wear, and not simply the “total duration” per day [ 166 , 167 , 168 , 169 ]. These findings argue for a nonlinear processing of myopic and hyperopic defocus signals across the retina [ 166 , 167 ].
4.3.3 Comparing Form-Deprivation and Lens Defocus
Although FD and lens induced defocus use different visual stimuli to induce compensatory changes in ocular growth, there are features that are common to both visual manipulations. Removal of the visual manipulation triggers “recovery” from both FD and lens induced defocus [ 16 , 17 , 170 ]. During this recovery phase, the eyes quickly return to emmetropia by reversing the changes in choroidal thickness and axial eye growth (mainly by changing the vitreous chamber depth) [ 16 , 17 , 119 , 150 , 151 , 170 , 171 , 172 , 173 ].
Further evidence from animal work suggests that the elimination of accommodation by cycloplegia, ciliary nerve section or damage to the Edinger-Westphal nucleus does not prevent the response to imposed FD [ 145 , 174 ] or lens induced defocus [ 148 , 175 ]. These results suggest that intact accommodation is not essential for visually guided growth [ 176 ] or there might be another accommodative pathway (not through ciliary and iris sphincter muscles) underlying optical defocus induced alterations in ocular growth [ 175 ].
Other studies argue that mechanisms underlying the response to FD and lens induced defocus may not be the same [ 177 ]. Some studies show different light paradigms selectively disrupt the response to FD or lens induced defocus [ 178 , 179 , 180 ]. For instance, high luminance levels inhibit myopia caused by FD in monkeys and chicks, but only slow the response to negative lenses [ 181 , 182 ]. Furthermore, dopamine (a strong ocular growth inhibitor, see Sect. 4.4.1 ) may not signal eye growth in a similar manner for these two forms of experimental myopia [ 183 ]. While most studies indicate that dopamine agonists block increased axial elongation [ 177 ], one study reported that dopamine agonists inhibit FD, but not lens-induced myopia in guinea pigs [ 184 ]. More studies are needed to determine if these contradictory results are due to different regulatory mechanisms of eye growth or other parameters of the experimental paradigm.
4.4 Other Visual Cues for Emmetropization
Whilst the clarity of the retinal image dominates the nature of ocular growth, other visual cues may also influence the process of emmetropization. This section examines some of the important cues that could significantly affect retinal image quality, and hence ocular growth in human eyes.
4.4.1 Retinal Physiology
Work from animal models suggests that retinal defocus (or visual blur) initiates a signaling cascade that leads to a number of cellular and biochemical changes in the retina and the retinal pigment epithelium (RPE), which signal changes to the choroid, and eventually the sclera, leading to alterations in the overall growth and refractive state of the eye (Fig. 4.7 ) [ 16 , 17 , 185 ]. The retina is an integral part of this visual signaling as it is the first layer of photosensory neurons that detect defocus [ 186 ]. Furthermore, ocular compensation for both FD and lens induced defocus are largely regulated at the retinal level. Severing the optic nerve in young chicks does not prevent the development of refractive errors in response to spectacle lenses [ 16 , 187 ] or diffusers [ 130 ]. In both chicks [ 17 , 188 , 189 ] and primates [ 190 ], partial diffusers and hemifield spectacle lenses restricted to only half of the visual field cause corresponding myopic changes only in the visually deprived part of the globe. These studies demonstrate that the visual regulation of ocular growth in response to diffusers and lenses primarily occurs within the retina, with minimal input from the brain.
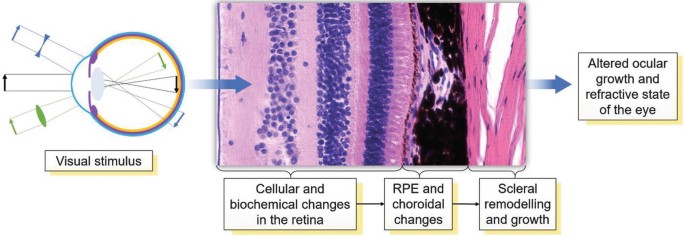
A biochemical signal cascade beginning at the retina and ending at the sclera regulating ocular growth and the refractive state. Retinal defocus may initiate a signaling cascade that leads to a number of cellular and biochemical changes in the retina and the retinal pigment epithelium (RPE), the choroid, and eventually the sclera, leading to alterations in the overall growth and the refractive state of the eye
Retinal neurons also secrete a number of growth regulatory neurotransmitters (such as dopamine [ 191 , 192 ], retinoic acid [ 153 ], nitric oxide [ 193 , 194 ] and glucagon [ 195 ]) that can directly alter ocular growth in mammalian eyes. Dopamine, one of the most widely studied neurotransmitters with regard to myopia in animal models, has been implicated as a potent stop signal for myopic eye growth [ 183 , 196 ]. In both chickens [ 191 , 197 ] and primates [ 198 ], FD myopia is associated with lower levels of 3,4-dihydroxyphenylacetate (DOPAC, the primary metabolite of dopamine) and dopamine in the retina. Although the protective effects of outdoor light exposure on myopia development in children has been hypothesized to be mediated by greater dopamine synthesis in the eye (see Sect. 4.5 ), the exact mechanisms underlying the protective effects of dopamine on myopia are not fully known. Together, these studies suggest that alterations in normal retinal physiology and/or changes in retinal neurotransmitters may lead to the development of refractive errors, as shown in chickens [ 186 , 199 , 200 ] and mice [ 201 , 202 , 203 , 204 , 205 , 206 ]. Some features of retinal abnormalities and refractive errors are evident in humans as well; for instance, NYX [ 207 ] and GRM6 [ 208 ] retinal ON pathway mutations and retinal degenerations such as cone-rod dystrophy [ 209 ] and retinitis pigmentosa [ 210 ] are associated with myopia.
4.4.2 Aberrations
A long held belief is that myopia may develop due to the eye’s emmetropization response to inherent ocular aberrations that degrade retinal image quality and trigger axial elongation [ 211 ]. Since there is minimal variation in longitudinal chromatic aberration between individuals or refractive error groups in humans [ 212 ], most investigations have focused on monochromatic higher order aberrations (HOAs) as a potential myopigenic stimulus. Evidence concerning the relationship between HOAs during distance viewing and refractive error from cross-sectional studies is conflicting [ 211 , 213 ]. However, during or following near work tasks, adult myopic eyes tend to display a transient increase in corneal and total ocular HOAs (Figs. 4.8 and 4.9 ), suggesting a potential role for near work induced retinal image degradation in myopia development [ 214 , 215 ]. Longitudinal studies of myopic children also indicate that eyes with greater positive spherical aberration demonstrate slower eye growth [ 63 , 216 ].
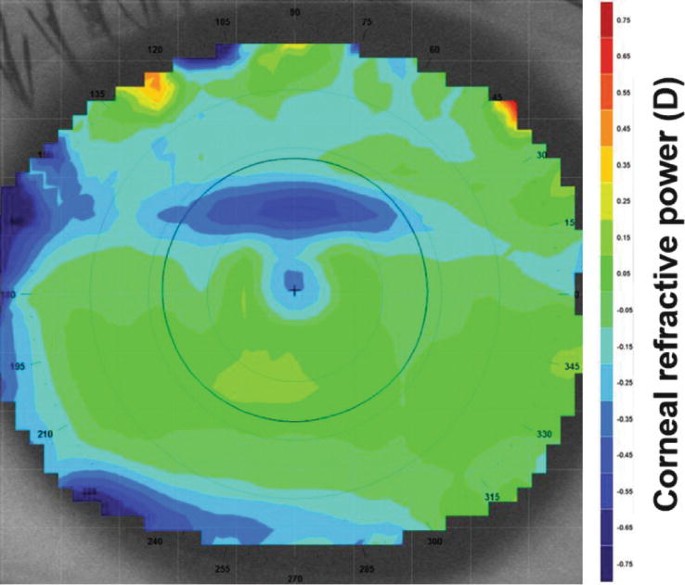
Corneal refractive power difference map following a 10 min reading task at 25° downward gaze. The black circle denotes the pupil outline detected by the video keratoscope. A horizontal band of corneal flattening is observed in the superior aspect of the pupil corresponding to the position of the upper eyelid during downward gaze. This refractive change is equivalent to a 0.20 D hyperopic shift over the central 4 mm
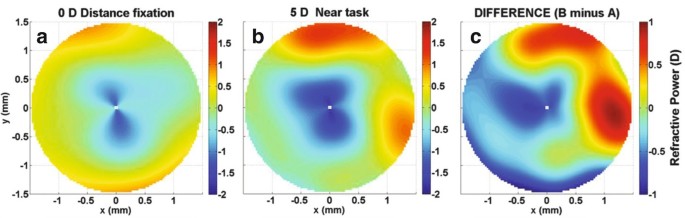
Refractive power maps, a graphical representation of total ocular higher order aberrations, during ( a ) distance fixation (0 D accommodation demand), ( b ) a 5 D accommodation task, and ( c ) the difference (B minus A) over a 3 mm pupil diameter. A significant increase in negative spherical aberration of −0.10 μm is displayed
A number of myopia control interventions also alter the HOA and peripheral refraction profile. While relative peripheral refraction was initially thought to modulate central eye growth, recent longitudinal studies have found no association between peripheral refraction and myopia progression in children [ 217 , 218 ]. Multifocal soft contact lenses and orthokeratology significantly increase the magnitude of positive spherical aberration [ 219 , 220 ]. The anticholinergic agent atropine may also provide visual feedback that influences eye growth due to an increase in positive spherical aberration or horizontal coma associated with cycloplegia and pupil mydriasis, respectively [ 221 ]. Collectively, these findings suggest that changes in HOAs may influence eye growth and refractive development during childhood.
4.4.3 Accommodation
Given the association between near work and the development and progression of childhood myopia [ 222 ], numerous studies have compared various characteristics of accommodation between refractive error groups, typically the accuracy of the accommodation response, since a lag of accommodation (hyperopic retinal defocus) may stimulate axial elongation as observed in animal models. Using a range of experimental approaches, the accommodative response to a 3 D accommodative stimulus is ~0.25 to 1.00 D less in myopic adults compared to emmetropes [ 223 , 224 , 225 , 226 , 227 ]. The slowing of myopia progression during childhood with progressive addition or bifocal lenses, designed to improve accommodation accuracy and minimize a lag of accommodation, adds some weight to the role of accommodation in myopia development and progression [ 62 , 228 ]. However, the exact underlying mechanism of myopia control with such lenses may be related to imposed peripheral retinal defocus or a reduction in the near vergence demand [ 229 ]. In a longitudinal study of young infants [ 230 ], a significant relationship was observed between the accommodative response and the reduction in neonatal refractive error in the first 2 years of life, supporting a potential role for accommodation-guided eye growth.
4.4.4 Circadian Rhythms
Like many of the human body’s physiological processes, numerous ocular structures and functions exhibit cyclic variations over the course of the day. Visual inputs such as daily patterns of light exposure are considered critical factors in entraining the timing of these circadian rhythms. Findings from animal studies demonstrate that normal eye growth exhibits significant circadian variations, with the eye generally being longest during the day and shorter at night [ 231 ]. Choroidal thickness also exhibits a circadian rhythm in normal eyes, which is generally in antiphase to the rhythms in axial length [ 172 ]. Similar patterns of diurnal variation in axial length and choroidal thickness have also been documented in normal human eyes [ 232 , 233 , 234 ].
It has also been suggested that ocular circadian rhythms may play a role in eye growth regulation and the development of myopia, since altering the visual inputs that drive circadian rhythms (e.g., rearing animals in constant light [ 235 ] or constant darkness [ 236 ], or exposing the eye to bright light at night time [ 237 ]) can result in alterations in normal eye growth in animal models. Furthermore, when refractive errors (both hyperopic and myopic) are induced experimentally in animals, changes in the magnitude and phase of the normal circadian rhythms of axial length and choroidal thickness also occur, which also supports a potential role of circadian rhythms in the development of refractive errors [ 172 , 238 ]. These findings from animal research were paralleled by studies in young adult humans, where changes in the normal diurnal rhythms of axial length and choroidal thickness occurred in response to short-term (12-h) exposure to monocular myopic [ 165 ], and hyperopic blur [ 163 ]. Although the exact role played by circadian rhythms in the regulation of human eye growth is not fully understood, human studies also indicate that myopes exhibit alterations in their systemic melatonin levels [ 239 ], and also exhibit altered sleep patterns [ 240 ] compared to non-myopic individuals.
4.5 Effects of Key Environmental Factors on Myopia
As discussed in Chaps. 1 and 2 , myopia represents a “complex” disorder with both environmental and genetic origins [ 241 , 242 ]. This section discusses some of the important environmental factors, and their influence on myopia.
4.5.1 Near Work and Education
Since the age when myopia normally develops and progresses coincides with the school years, myopia has long been suggested to be connected with increased levels of education. Indeed, numerous studies conducted across a range of different populations have consistently found that higher levels of education are associated with a higher prevalence of myopia [ 243 , 244 , 245 ]. The exact mechanism linking increased education with myopia, however, is less clear. Although it is possible that optical [ 214 , 224 ] or biomechanical [ 246 , 247 ] ocular changes associated with near work could potentially promote myopic eye growth in those with higher levels of education (and hence near work demands), population studies examining the link between near work activities and myopia have been conflicting, with some studies suggesting an association between near work and myopia [ 222 , 248 ], and others indicating no significant effects [ 249 ]. The relatively inconsistent findings linking near work with myopia development suggests a potential role for other factors in the association between education and myopia, such as a lack of outdoor light exposure, discussed further below.
4.5.2 Urbanization
Aspects of the living environment may also be involved in the development and progression of myopia, since population-based studies consistently report a higher prevalence of myopia in children living in urban regions, compared to children living in rural regions [ 250 , 251 ]. These associations between the urban environment and myopia could at least partially be explained by socioeconomic and educational differences between urban and rural regions, which could in turn result in differences in near work and outdoor activities. However, a number of recent studies indicate that a higher population density is significantly associated with increased myopia prevalence in children, independent of near work and outdoor activities [ 252 , 253 ]. This suggests other aspects of the urban environment may potentially impact upon eye growth. Studies have also reported associations between housing type and myopia, with children living in smaller homes reported to have a significantly higher prevalence of myopia [ 253 , 254 ]. Although further research is required to establish the causative nature and mechanisms underlying these associations, it has been hypothesized that a constricted living space may result in an increased exposure to hyperopic blur, thus promoting myopia.
4.5.3 Light Exposure
A number of recent studies report that the time children spend engaged in outdoor activities is negatively associated with their risk of myopia [ 241 , 249 , 255 , 256 , 257 , 258 , 259 ]. Both cross-sectional and longitudinal studies indicate that greater time spent outdoors is associated with a significantly lower myopia prevalence and reduced risk of myopia onset in childhood. Although some studies report significant associations between myopia progression and outdoor activity [ 257 , 259 ], this is not a consistent finding across all longitudinal studies [ 260 ]. A recent meta-analysis of studies examining the relationship between outdoor time and myopia indicated that there was a 2% reduction in the odds of having myopia for each additional hour per week spent outdoors [ 261 ].
These associations [ 241 , 255 , 256 ] have prompted recent interest in the potential influence of light exposure in the regulation of eye growth and myopia. Since outdoor activity typically involves exposure to high intensity light, it has been hypothesized that increased exposure to bright light may be the important factor underlying these protective effects of outdoor activity [ 256 ]. Other factors, such as the typical pattern of retinal focus experienced in outdoor environments (which is likely to involve less near focusing and potentially less exposure to hyperopic blur), may also play a role [ 262 ].
Light Intensity and Myopia
Animal studies indicate that the intensity of daily light exposure can influence refractive development. In normal growing young chickens, rearing animals under a normal daily light-dark cycle, but with daily bright ambient lighting (~10,000 lux) resulted in significantly less myopic refractive errors than when animals were reared under dim ambient lighting during the day (50 lux) [ 263 ]. Bright light exposure also inhibits the development of FD myopia in a range of different animal species [ 114 , 181 , 264 ], with the strength of inhibitory effects correlating significantly with the log of the intensity of ambient light exposure [ 264 ]. The effects of increased light intensity upon lens induced myopia (through imposing hyperopic defocus) in animals however are less consistent, with either a slowing of lens compensation (with no change in refractive endpoint) [ 182 ], or no significant effects on lens compensation reported [ 265 ].
Recent observational longitudinal studies in humans utilizing wearable light sensors to assess ambient light exposure have enabled the relationship between light exposure and axial eye growth in childhood to be examined [ 266 , 267 ]. Similar to findings in animals, both of these recent studies have reported that slower axial eye growth is associated with greater daily ambient light exposure (with a 1-log unit increase in light exposure being associated with ~0.1 mm/year slower axial eye growth), with this relationship reaching statistical significance in the study with the larger sample size ( n = 102) [ 266 ], and bordering on significance ( p = 0.07, n = 60) in the other study [ 267 ]. A recent randomized, controlled trial in Taiwan examined the effect of increasing outdoor time during the school day (an extra 40 min of outdoor time during school recess) upon myopia development and axial eye growth over 12 months [ 268 ]. In this study, light exposure was also monitored using wearable light sensors, and a significant association between greater light exposure and slower myopia progression was also documented. Collectively, these studies suggest that increased light exposure is associated with slower axial eye growth in the human eye.
Increased light exposure may also underlie some of the differences in myopia prevalence found in different geographic locations. A recent study compared the habitual ambient light exposure (captured with wearable light sensors) of children living in Singapore (a country with some of the highest reported levels of childhood myopia prevalence [ 269 ]) with children living in Australia (where myopia prevalence is generally reported to be relatively low [ 270 ]) and found substantially lower levels of outdoor light exposure in the children living in Singapore (Fig. 4.10 ) [ 271 ].

Average hourly light exposure of Australian (red lines) and Singaporean (blue lines) children assessed during school weekdays using wearable light sensors. Note the substantially greater light exposure for the Australian children at a number of periods throughout the day [ 271 ]. This lower daily exposure to bright outdoor light may be one factor underlying the higher myopia prevalence typically observed in Singaporean children. Dashed lines indicate the average school start and finish times in Australia (red) and Singapore (blue). Error bars represent the standard error of the mean
Duration of Light Exposure and Myopia
Animal studies examining the effects of increased light exposure upon myopia development have generally used experimental paradigms where elevated light levels were applied continuously for the full day. Lan et al. [ 272 ] examined the influence of different daily durations of bright light exposure upon inhibition of myopia in chickens. They found bright light applied for only 1 or 2 h per day did not inhibit myopia, but 5 h of exposure did significantly protect against the development of FD myopia. Extending exposure duration further to 10 h per day did not appear to offer further protective benefit.
In a large longitudinal study, Jones et al. [ 249 ] reported that children who engaged in outdoor activities for 14 h per week or more, exhibited the lowest odds of developing myopia. A number of recent randomized controlled trials have reported that interventions that increase children’s outdoor time (by 40–80 min a day) significantly reduce the onset of myopia in childhood [ 268 , 273 , 274 ]. In the “Role of outdoor activity in myopia study” [ 266 ], children who were habitually exposed to low ambient light levels (on average less than 60 min exposure to outdoor light per day) had significantly faster axial eye growth compared to children habitually exposed to moderate and high light levels. These findings from human studies suggest that children who are exposed to less than 60 min a day of bright outdoor light are at an increased risk of more rapid eye growth and myopia development, and that approximately 2 h or more of outdoor exposure each day is required to provide protection against myopia development in the human eye.
Spectral Composition of Light and Myopia
Since the spectral characteristics of outdoor light are significantly different to typical indoor light, it has been suggested that the spectral composition of outdoor light may be an additional factor that underlies the protective effects of outdoor activity upon myopia development. Although some studies in humans suggest exposure to short-wavelength light may protect against myopia [ 275 ], there has only been limited work in humans examining the possible impact of the spectral composition of light on myopia.
Animal studies do suggest that the spectral content of light can influence the growth of the eye, since altered eye growth is observed in animals reared under narrow band spectral lighting conditions. However, the effects of the spectral content of light shows substantial interspecies differences [ 276 , 277 , 278 , 279 ]. In chickens and guineas pigs, raising animals in short-wavelength light appears to slow eye growth and reduce myopia development, whereas long-wavelength light appears to increase eye growth and lead to myopia development [ 276 , 279 ]. Conversely long-wavelength light appears to slow eye growth and reduce myopia development in tree shrews and rhesus monkeys [ 277 , 278 ]. Further research is required to understand the mechanisms underlying these effects (and interspecies differences) and to establish the impact of the spectral composition of light upon human myopia.
4.6 Conclusion
In conclusion, over the last 40 years, remarkable progress has been made in understanding the possible mechanisms and pathogenesis of myopia, with a large contribution to this knowledge coming from an extensive body of work in animal models. Importantly, laboratory research in animals have shown that the visual environment (i.e., quality and/or focus of the retinal image) influences ocular growth and refractive development, which has been a key to our current understanding of the process of emmetropization in humans. Similarities in features of defocus induced ocular changes in humans and experimental models of myopia, such as the eye’s ability to detect the sign of retinal defocus and make compensatory changes in axial length, suggest that mechanisms of visually guided eye growth and refractive error development in animal models may be present in human eyes as well. Alongside animal research, a large body of clinical and epidemiological research has identified a number of other visual cues (e.g., aberrations, accommodation, and circadian rhythms) and environmental factors (e.g., light exposure, near work, and education) that could affect normal ocular growth and lead to the development of refractive errors. Experimental models continue to provide valuable information on cellular and biochemical mechanisms of eye growth, enabling the identification of potential new therapeutic targets for early diagnosis and treatment of myopia.
Wallman J, Winawer J. Homeostasis of eye growth and the question of myopia. Neuron. 2004;43:447–68.
CAS PubMed Google Scholar
Smith EL. Spectacle lenses and emmetropization: the role of optical defocus in regulating ocular development. Optom Vis Sci. 1998;75:388–98.
PubMed Google Scholar
Cook RC, Glasscock R. Refractive and ocular findings in the newborn. Am J Ophthalmol. 1951;34:1407–13.
Banks MS. Infant refraction and accommodation. Int Ophthalmol Clin. 1980;20:205–32.
Mohindra I, Held R. Refraction in humans from birth to five years. In: Third International Conference on Myopia Copenhagen, August 24–27, 1980. New York: Springer; 1981. p. 19–27.
Google Scholar
Ingram R, Barr A. Changes in refraction between the ages of 1 and 3 1/2 years. Br J Ophthalmol. 1979;63:339–42.
CAS PubMed PubMed Central Google Scholar
Wood I, Hodi S, Morgan L. Longitudinal change of refractive error in infants during the first year of life. Eye. 1995;9:551–7.
Gwiazda J, Thorn F, Bauer J, Held R. Emmetropization and the progression of manifest refraction in children followed from infancy to puberty. Clin Vis Sci. 1993;8:337–44.
Kempf GA, Collins SD, Jarman BL. Refractive errors in the eyes of children as determined by retinoscopie examination with a cycloplegic. Results of eye examinations of 1,860 white school children in Washington, DC. Public Health Bulletin. 1928;182:56.
Fitzgerald DE, Duckman R. Refractive error. In: Visual development, diagnosis, and treatment of the pediatric patient. Philadelphia: Lippincott Williams & Wilkins; 2006. p. 69–88.
Gordon RA, Donzis PB. Refractive development of the human eye. Arch Ophthalmol. 1985;103:785–9.
Saunders KJ. Early refractive development in humans. Surv Ophthalmol. 1995;40:207–16.
Hirsch MJ. Predictability of refraction at age 14 on the basis of testing at age 6—interim report from the Ojai Longitudinal Study of Refraction. Optom Vis Sci. 1964;41:567–73.
CAS Google Scholar
Zadnik K, Mutti DO, Friedman NE, Adams AJ. Initial cross-sectional results from the Orinda Longitudinal Study of Myopia. Optom Vis Sci. 1993;70:750–8.
Smith EL, Hung LF. The role of optical defocus in regulating refractive development in infant monkeys. Vis Res. 1999;39:1415–35.
Wildsoet C, Wallman J. Choroidal and scleral mechanisms of compensation for spectacle lenses in chicks. Vis Res. 1995;35:1175–94.
Wallman J, Wildsoet C, Xu A, et al. Moving the retina: choroidal modulation of refractive state. Vis Res. 1995;35:37–50.
Mutti DO, Mitchell GL, Jones LA, et al. Axial growth and changes in lenticular and corneal power during emmetropization in infants. Invest Ophthalmol Vis Sci. 2005;46:3074–80.
Pennie FC, Wood IC, Olsen C, White S, Charman WN. A longitudinal study of the biometric and refractive changes in full-term infants during the first year of life. Vis Res. 2001;41:2799–810.
Larsen JS. The sagittal growth of the eye: IV. Ultrasonic measurement of the axial length of the eye from birth to puberty. Acta Ophthalmol. 1971;49:873–86.
Larsen JS. The sagittal growth of the eye. 3. Ultrasonic measurement of the posterior segment (axial length of the vitreous) from birth to puberty. Acta Ophthalmol. 1971;49:441–53.
Sorsby A, Leary G, Richards MJ. Correlation ametropia and component ametropia. Vis Res. 1962;2:309–13.
Isenberg SJ, Del Signore M, Chen A, Wei J, Christenson PD. Corneal topography of neonates and infants. Arch Ophthalmol. 2004;122:1767–71.
Ehrlich DL, Braddick OJ, Atkinson J, et al. Infant emmetropization: longitudinal changes in refraction components from nine to twenty months of age. Optom Vis Sci. 1997;74:822–43.
Varughese S, Varghese RM, Gupta N, Ojha R, Sreenivas V, Puliyel JM. Refractive error at birth and its relation to gestational age. Curr Eye Res. 2005;30:423–8.
Friling R, Weinberger D, Kremer I, Avisar R, Sirota L, Snir M. Keratometry measurements in preterm and full term newborn infants. Br J Ophthalmol. 2004;88:8–10.
Rowland HC, Sayles N. Photokeratometric and photorefractive measurements of astigmatism in infants and young children. Vis Res. 1985;25:73–81.
Gwiazda J, Scheiman M, Mohindra I, Held R. Astigmatism in children: changes in axis and amount from birth to six years. Invest Ophthalmol Vis Sci. 1984;25:88–92.
Atkinson J, Braddick O, French J. Infant astigmatism: its disappearance with age. Vis Res. 1980;20:891–3.
Dobson V, Fulton A, Sebris SL. Cycloplegic refractions of infants and young children: the axis of astigmatism. Invest Ophthalmol Vis Sci. 1984;25:83–7.
Li SM, Iribarren R, Kang MT, et al. Corneal power, anterior segment length and lens power in 14-year-old Chinese children: the Anyang Childhood Eye Study. Sci Rep. 2016;6:20243.
Carney LG, Mainstone JC, Henderson BA. Corneal topography and myopia. A cross-sectional study. Invest Ophthalmol Vis Sci. 1997;38:311–20.
AlMahmoud T, Priest D, Munger R, Jackson WB. Correlation between refractive error, corneal power, and thickness in a large population with a wide range of ametropia. Invest Ophthalmol Vis Sci. 2011;52:1235–42.
Xie R, Zhou XT, Lu F, et al. Correlation between myopia and major biometric parameters of the eye: a retrospective clinical study. Optom Vis Sci. 2009;86:E503–8.
Zhang YY, Jiang WJ, Teng ZE, et al. Corneal curvature radius and associated factors in Chinese children: the Shandong Children Eye Study. PLoS One. 2015;10:e0117481.
PubMed PubMed Central Google Scholar
Breslin KM, O’Donoghue L, Saunders KJ. A prospective study of spherical refractive error and ocular components among Northern Irish schoolchildren (the NICER study). Invest Ophthalmol Vis Sci. 2013;54:4843–50.
Saw SM, Chua WH, Gazzard G, Koh D, Tan DT, Stone RA. Eye growth changes in myopic children in Singapore. Br J Ophthalmol. 2005;89:1489–94.
Scheiman M, Gwiazda J, Zhang Q, et al. Longitudinal changes in corneal curvature and its relationship to axial length in the Correction of Myopia Evaluation Trial (COMET) cohort. J Opt. 2016;9:13–21.
Lin LL, Shih YF, Lee YC, Hung PT, Hou PK. Changes in ocular refraction and its components among medical students--a 5-year longitudinal study. Optom Vis Sci. 1996;73:495–8.
Ojaimi E, Rose KA, Morgan IG, et al. Distribution of ocular biometric parameters and refraction in a population-based study of Australian children. Invest Ophthalmol Vis Sci. 2005;46:2748–54.
He X, Zou H, Lu L, et al. Axial length/corneal radius ratio: association with refractive state and role on myopia detection combined with visual acuity in Chinese schoolchildren. PLoS One. 2015;10:e0111766.
Gonzalez Blanco F, Sanz Fernandez JC, Munoz Sanz MA. Axial length, corneal radius, and age of myopia onset. Optom Vis Sci. 2008;85:89–96.
Foo VH, Verkicharla PK, Ikram MK, et al. Axial length/corneal radius of curvature ratio and myopia in 3-year-old children. Transl Vis Sci Technol. 2016;5:5.
Ortiz S, Mena L, Rio-San Cristobal A, Martin R. Relationships between central and peripheral corneal thickness in different degrees of myopia. J Opt. 2014;7:44–50.
Pedersen L, Hjortdal J, Ehlers N. Central corneal thickness in high myopia. Acta Ophthalmol Scand. 2005;83:539–42.
Tong L, Saw SM, Siak JK, Gazzard G, Tan D. Corneal thickness determination and correlates in Singaporean schoolchildren. Invest Ophthalmol Vis Sci. 2004;45:4004–9.
Fam HB, How AC, Baskaran M, Lim KL, Chan YH, Aung T. Central corneal thickness and its relationship to myopia in Chinese adults. Br J Ophthalmol. 2006;90:1451–3.
Huang Y, Huang C, Li L, et al. Corneal biomechanics, refractive error, and axial length in Chinese primary school children. Invest Ophthalmol Vis Sci. 2011;52:4923–8.
Chang PY, Chang SW, Wang JY. Assessment of corneal biomechanical properties and intraocular pressure with the Ocular Response Analyzer in childhood myopia. Br J Ophthalmol. 2010;94:877–81.
Jiang Z, Shen M, Mao G, et al. Association between corneal biomechanical properties and myopia in Chinese subjects. Eye. 2011;25:1083–9.
Wang W, He M, He H, Zhang C, Jin H, Zhong X. Corneal biomechanical metrics of healthy Chinese adults using Corvis ST. Cont Lens Anterior Eye. 2017;40:97–103.
Shen M, Fan F, Xue A, Wang J, Zhou X, Lu F. Biomechanical properties of the cornea in high myopia. Vis Res. 2008;48:2167–71.
Shih YF, Chiang TH, Lin LL. Lens thickness changes among school children in Taiwan. Invest Ophthalmol Vis Sci. 2009;50:2637–44.
Wong HB, Machin D, Tan SB, Wong TY, Saw SM. Ocular component growth curves among Singaporean children with different refractive error status. Invest Ophthalmol Vis Sci. 2010;51:1341–7.
Jones LA, Mitchell GL, Mutti DO, Hayes JR, Moeschberger ML, Zadnik K. Comparison of ocular component growth curves among refractive error groups in children. Invest Ophthalmol Vis Sci. 2005;46:2317–27.
Mutti DO, Mitchell GL, Sinnott LT, et al. Corneal and crystalline lens dimensions before and after myopia onset. Optom Vis Sci. 2012;89:251–62.
Iribarren R, Morgan IG, Chan YH, Lin X, Saw SM. Changes in lens power in Singapore Chinese children during refractive development. Invest Ophthalmol Vis Sci. 2012;53:5124–30.
Dubbelman M, Van der Heijde GL. The shape of the aging human lens: curvature, equivalent refractive index and the lens paradox. Vis Res. 2001;41:1867–77.
Li SM, Li SY, Kang MT, et al. Distribution of ocular biometry in 7- and 14-year-old Chinese children. Optom Vis Sci. 2015;92:566–72.
Richter GM, Wang M, Jiang X, et al. Ocular determinants of refractive error and its age- and sex-related variations in the Chinese American Eye Study. JAMA Ophthalmol. 2017;135:724–32.
Zadnik K, Mutti DO, Mitchell GL, Jones LA, Burr D, Moeschberger ML. Normal eye growth in emmetropic schoolchildren. Optom Vis Sci. 2004;81:819–28.
Gwiazda J, Hyman L, Hussein M, et al. A randomized clinical trial of progressive addition lenses versus single vision lenses on the progression of myopia in children. Invest Ophthalmol Vis Sci. 2003;44:1492–500.
Lau JK, Vincent SJ, Collins MJ, Cheung SW, Cho P. Ocular higher-order aberrations and axial eye growth in young Hong Kong children. Sci Rep. 2018;8:6726.
Huang J, Wen D, Wang Q, et al. Efficacy comparison of 16 interventions for myopia control in children: a network meta-analysis. Ophthalmology. 2016;123:697–708.
Read SA, Collins MJ, Vincent SJ, Alonso-Caneiro D. Choroidal thickness in myopic and nonmyopic children assessed with enhanced depth imaging optical coherence tomography. Invest Ophthalmol Vis Sci. 2013;54:7578–86.
Xiong S, He X, Deng J, et al. Choroidal thickness in 3001 Chinese children aged 6 to 19 years using swept-source OCT. Sci Rep. 2017;7:45059.
Chen FK, Yeoh J, Rahman W, Patel PJ, Tufail A, Da Cruz L. Topographic variation and interocular symmetry of macular choroidal thickness using enhanced depth imaging optical coherence tomography. Invest Ophthalmol Vis Sci. 2012;53:975–85.
Esmaeelpour M, Povazay B, Hermann B, et al. Three-dimensional 1060-nm OCT: choroidal thickness maps in normal subjects and improved posterior segment visualization in cataract patients. Invest Ophthalmol Vis Sci. 2010;51:5260–6.
Gupta P, Jing T, Marziliano P, et al. Distribution and determinants of choroidal thickness and volume using automated segmentation software in a population-based study. Am J Ophthalmol. 2015;159:293–301.e293.
Li XQ, Munkholm A, Larsen M, Munch IC. Choroidal thickness in relation to birth parameters in 11- to 12-year-old children: the Copenhagen Child Cohort 2000 Eye Study. Invest Ophthalmol Vis Sci. 2014;56:617–24.
Ouyang Y, Heussen FM, Mokwa N, et al. Spatial distribution of posterior pole choroidal thickness by spectral domain optical coherence tomography. Invest Ophthalmol Vis Sci. 2011;52:7019–26.
Tan CS, Cheong KX. Macular choroidal thicknesses in healthy adults--relationship with ocular and demographic factors. Invest Ophthalmol Vis Sci. 2014;55:6452–8.
Wei WB, Xu L, Jonas JB, et al. Subfoveal choroidal thickness: the Beijing Eye Study. Ophthalmology. 2013;120:175–80.
Sanchez-Cano A, Orduna E, Segura F, et al. Choroidal thickness and volume in healthy young white adults and the relationships between them and axial length, ammetropy and sex. Am J Ophthalmol. 2014;158:574–83.
He X, Jin P, Zou H, et al. Choroidal thickness in healthy Chinese children aged 6 to 12: The Shanghai Children Eye Study. Retina. 2017;37:368–75.
Jin P, Zou H, Zhu J, et al. Choroidal and retinal thickness in children with different refractive status measured by swept-source optical coherence tomography. Am J Ophthalmol. 2016;168:164–76.
Zhang JM, Wu JF, Chen JH, et al. Macular choroidal thickness in children: the Shandong Children Eye Study. Invest Ophthalmol Vis Sci. 2015;56:7646–52.
Zhou LX, Shao L, Xu L, Wei WB, Wang YX, You QS. The relationship between scleral staphyloma and choroidal thinning in highly myopic eyes: the Beijing Eye Study. Sci Rep. 2017;7:9825.
Wang NK, Lai CC, Chou CL, et al. Choroidal thickness and biometric markers for the screening of lacquer cracks in patients with high myopia. PLoS One. 2013;8:e53660.
Ikuno Y, Jo Y, Hamasaki T, Tano Y. Ocular risk factors for choroidal neovascularization in pathologic myopia. Invest Ophthalmol Vis Sci. 2010;51:3721–5.
Nishida Y, Fujiwara T, Imamura Y, Lima LH, Kurosaka D, Spaide RF. Choroidal thickness and visual acuity in highly myopic eyes. Retina. 2012;32:1229–36.
Read SA, Alonso-Caneiro D, Vincent SJ, Collins MJ. Longitudinal changes in choroidal thickness and eye growth in childhood. Invest Ophthalmol Vis Sci. 2015;56:3103–12.
Fontaine M, Gaucher D, Sauer A, Speeg-Schatz C. Choroidal thickness and ametropia in children: a longitudinal study. Eur J Ophthalmol. 2017;27:730–4.
Jin P, Zou H, Xu X, et al. Longitudinal changes in choroidal and retinal thicknesses in children with myopic shift. Retina. 2019;39:1091–9.
Chen Z, Xue F, Zhou J, Qu X, Zhou X. Effects of orthokeratology on choroidal thickness and axial length. Optom Vis Sci. 2016;93:1064–71.
Li Z, Cui D, Hu Y, Ao S, Zeng J, Yang X. Choroidal thickness and axial length changes in myopic children treated with orthokeratology. Cont Lens Anterior Eye. 2017;40:417–23.
Sander BP, Collins MJ, Read SA. The effect of topical adrenergic and anticholinergic agents on the choroidal thickness of young healthy adults. Exp Eye Res. 2014;128:181–9.
Zhang Z, Zhou Y, Xie Z, et al. The effect of topical atropine on the choroidal thickness of healthy children. Sci Rep. 2016;6:34936.
Read SA. Ocular and environmental factors associated with eye growth in childhood. Optom Vis Sci. 2016;93:1031–41.
Elsheikh A, Geraghty B, Alhasso D, Knappett J, Campanelli M, Rama P. Regional variation in the biomechanical properties of the human sclera. Exp Eye Res. 2010;90:624–33.
Norman RE, Flanagan JG, Rausch SM, et al. Dimensions of the human sclera: thickness measurement and regional changes with axial length. Exp Eye Res. 2010;90:277–84.
Vurgese S, Panda-Jonas S, Jonas JB. Scleral thickness in human eyes. PLoS One. 2012;7:e29692.
McBrien NA, Gentle A. Role of the sclera in the development and pathological complications of myopia. Prog Retin Eye Res. 2003;22:307–38.
Downs JC, Ensor ME, Bellezza AJ, Thompson HW, Hart RT, Burgoyne CF. Posterior scleral thickness in perfusion-fixed normal and early-glaucoma monkey eyes. Invest Ophthalmol Vis Sci. 2001;42:3202–8.
Nemeth J. The posterior coats of the eye in glaucoma. An echobiometric study. Graefes Arch Clin Exp Ophthalmol. 1990;228:33–5.
Xue A, Bao F, Zheng L, Wang Q, Cheng L, Qu J. Posterior scleral reinforcement on progressive high myopic young patients. Optom Vis Sci. 2014;91:412–8.
Xue A, Zheng L, Tan G, et al. Genipin-crosslinked donor sclera for posterior scleral contraction/reinforcement to fight progressive myopia. Invest Ophthalmol Vis Sci. 2018;59:3564–73.
Read SA, Alonso-Caneiro D, Vincent SJ, et al. Anterior eye tissue morphology: scleral and conjunctival thickness in children and young adults. Sci Rep. 2016;6:33796.
Buckhurst HD, Gilmartin B, Cubbidge RP, Logan NS. Measurement of scleral thickness in humans using anterior segment optical coherent tomography. PLoS One. 2015;10:e0132902.
Pekel G, Yagci R, Acer S, Ongun GT, Cetin EN, Simavli H. Comparison of corneal layers and anterior sclera in emmetropic and myopic eyes. Cornea. 2015;34:786–90.
Consejo A, Radhakrishnan H, Iskander DR. Scleral changes with accommodation. Ophthalmic Physiol Opt. 2017;37:263–74.
Woodman-Pieterse EC, Read SA, Collins MJ, Alonso-Caneiro D. Anterior scleral thickness changes with accommodation in myopes and emmetropes. Exp Eye Res. 2018;177:96–103.
Bailey MD, Sinnott LT, Mutti DO. Ciliary body thickness and refractive error in children. Invest Ophthalmol Vis Sci. 2008;49:4353–60.
Kuchem MK, Sinnott LT, Kao CY, Bailey MD. Ciliary muscle thickness in anisometropia. Optom Vis Sci. 2013;90:1312–20.
Muftuoglu O, Hosal BM, Zilelioglu G. Ciliary body thickness in unilateral high axial myopia. Eye. 2009;23:1176–81.
Phillips JR, Khalaj M, McBrien NA. Induced myopia associated with increased scleral creep in chick and tree shrew eyes. Invest Ophthalmol Vis Sci. 2000;41:2028–34.
Siegwart JT Jr, Norton TT. Regulation of the mechanical properties of tree shrew sclera by the visual environment. Vis Res. 1999;39:387–407.
Sherman SM, Norton TT, Casagrande VA. Myopia in the lid-sutured tree shrew (Tupaia glis). Brain Res. 1977;124:154–7.
Wallman J, Turkel J, Trachtman J. Extreme myopia produced by modest change in early visual experience. Science. 1978;201:1249–51.
Wilson J, Sherman S. Differential effects of early monocular deprivation on binocular and monocular segments of cat striate cortex. J Neurophysiol. 1977;40:891–903.
Hodos W, Kuenzel WJ. Retinal-image degradation produces ocular enlargement in chicks. Invest Ophthalmol Vis Sci. 1984;25:652–9.
Wallman J, Ledoux C, Friedman MB. Simple devices for restricting the visual fields of birds. Behav Res Methods Instrum. 1978;10:401–3.
Howlett MH, McFadden SA. Form-deprivation myopia in the guinea pig (Cavia porcellus). Vis Res. 2006;46:267–83.
Ashby R, Ohlendorf A, Schaeffel F. The effect of ambient illuminance on the development of deprivation myopia in chicks. Invest Ophthalmol Vis Sci. 2009;50:5348–54.
Schaeffel F, Howland HC. Properties of the feedback loops controlling eye growth and refractive state in the chicken. Vis Res. 1991;31:717–34.
Schmid KL, Wildsoe CF. Contrast and spatial-frequency requirements for emmetropization in chicks. Vis Res. 1997;37:2011–21.
Wiesel TN, Raviola E. Myopia and eye enlargement after neonatal lid fusion in monkeys. Nature. 1977;266(5597):66–8.
Wildsoet CF, Schmid KL. Optical correction of form deprivation myopia inhibits refractive recovery in chick eyes with intact or sectioned optic nerves. Vis Res. 2000;40:3273–82.
Siegwart JT Jr, Norton TT. The susceptible period for deprivation-induced myopia in tree shrew. Vis Res. 1998;38:3505–15.
Troilo D, Nickla DL. The response to visual form deprivation differs with age in marmosets. Invest Ophthalmol Vis Sci. 2005;46:1873–81.
Smith EL, Hung L-F, C-s K, Qiao Y. Effects of brief periods of unrestricted vision on the development of form-deprivation myopia in monkeys. Invest Ophthalmol Vis Sci. 2002;43:291–9.
Smith EL, Li-Fang H, Harwerth RS. Effects of optically induced blur on the refractive status of young monkeys. Vis Res. 1994;34:293–301.
Schaeffel F, Feldkaemper M. Animal models in myopia research. Clin Exp Optom. 2015;98:507–17.
McBrien NA, Lawlor P, Gentle A. Scleral remodeling during the development of and recovery from axial myopia in the tree shrew. Invest Ophthalmol Vis Sci. 2000;41:3713–9.
Troilo D, Nickla DL, Wildsoet CF. Choroidal thickness changes during altered eye growth and refractive state in a primate. Invest Ophthalmol Vis Sci. 2000;41:1249–58.
Gottlieb MD, Joshi HB, Nickla DL. Scleral changes in chicks with form-deprivation myopia. Curr Eye Res. 1990;9:1157–65.
Hung LF, Wallman J, Smith EL. Vision-dependent changes in the choroidal thickness of macaque monkeys. Invest Ophthalmol Vis Sci. 2000;41:1259–69.
Funata M, Tokoro T. Scleral change in experimentally myopic monkeys. Graefes Arch Clin Exp Ophthalmol. 1990;228:174–9.
Smith EL 3rd, Hung LF. Form-deprivation myopia in monkeys is a graded phenomenon. Vis Res. 2000;40:371–81.
Troilo D, Gottlieb MD, Wallman J. Visual deprivation causes myopia in chicks with optic nerve section. Curr Eye Res. 1987;6:993–9.
Norton TT, Rada JA. Reduced extracellular matrix in mammalian sclera with induced myopia. Vis Res. 1995;35:1271–81.
Gottlieb MD, Fugate-Wentzek LA, Wallman J. Different visual deprivations produce different ametropias and different eye shapes. Invest Ophthalmol Vis Sci. 1987;28:1225–35.
Troilo D, Li T, Glasser A, Howland HC. Differences in eye growth and the response to visual deprivation in different strains of chicken. Vis Res. 1995;35:1211–6.
McKanna JA, Casagrande VA. Reduced lens development in lid-suture myopia. Exp Eye Res. 1978;26:715–23.
Napper GA, Brennan NA, Barrington M, Squires MA, Vessey GA, Vingrys AJ. The duration of normal visual exposure necessary to prevent form deprivation myopia in chicks. Vis Res. 1995;35:1337–44.
O’Leary D, Millodot M. Eyelid closure causes myopia in humans. Experientia. 1979;35:1478–9.
von Noorden GK, Lewis RA. Ocular axial length in unilateral congenital cataracts and blepharoptosis. Invest Ophthalmol Vis Sci. 1987;28:750–2.
Gee SS, Tabbara KF. Increase in ocular axial length in patients with corneal opacification. Ophthalmology. 1988;95:1276–8.
Miller-Meeks MJ, Bennett SR, Keech RV, Blodi CF. Myopia induced by vitreous hemorrhage. Am J Ophthalmol. 1990;109:199–203.
Bartmann M, Schaeffel F. A simple mechanism for emmetropization without cues from accommodation or colour. Vis Res. 1994;34:873–6.
Papastergiou GI, Schmid GF, Laties AM, Pendrak K, Lin T, Stone RA. Induction of axial eye elongation and myopic refractive shift in one-year-old chickens. Vis Res. 1998;38:1883–8.
Wildsoet C, Anchong R, Manasse J, Troilo D. Susceptibility to experimental myopia declines with age in the chick. Optom Vis Sci. 1998;75:265.
Bradley D, Fernandes A, Boothe R. Form deprivation myopia in adolescent monkeys. Optom Vis Sci. 1999;76:428–32.
Troilo D, Nickla DL, Wildsoet CF. Form deprivation myopia in mature common marmosets (Callithrix jacchus). Invest Ophthalmol Vis Sci. 2000;41:2043–9.
Schaeffel F, Glasser A, Howland HC. Accommodation, refractive error and eye growth in chickens. Vis Res. 1988;28:639–57.
Irving EL, Callender MG, Sivak JG. Inducing myopia, hyperopia, and astigmatism in chicks. Optom Vis Sci. 1991;68:364–8.
Irving EL, Sivak JG, Callender MG. Refractive plasticity of the developing chick eye. Ophthalmic Physiol Opt. 1992;12:448–56.
Schmid KL, Wildsoet CF. Effects on the compensatory responses to positive and negative lenses of intermittent lens wear and ciliary nerve section in chicks. Vis Res. 1996;36:1023–36.
Siegwart J, Norton T. Refractive and ocular changes in tree shrews raised with plus or minus lenses. Invest Ophthalmol Vis Sci. 1993;34:1208.
Hung LF, Crawford ML, Smith EL. Spectacle lenses alter eye growth and the refractive status of young monkeys. Nat Med. 1995;1:761–5.
Graham B, Judge SJ. The effects of spectacle wear in infancy on eye growth and refractive error in the marmoset (Callithrix jacchus). Vis Res. 1999;39:189–206.
Howlett MH, McFadden SA. Spectacle lens compensation in the pigmented guinea pig. Vis Res. 2009;49:219–27.
McFadden SA, Howlett MH, Mertz JR. Retinoic acid signals the direction of ocular elongation in the guinea pig eye. Vis Res. 2004;44:643–53.
Barathi VA, Boopathi VG, Yap EP, Beuerman RW. Two models of experimental myopia in the mouse. Vis Res. 2008;48:904–16.
Metlapally S, McBrien NA. The effect of positive lens defocus on ocular growth and emmetropization in the tree shrew. J Vis. 2008;8:1–12.
McFadden S, Wallman J. Guinea-pig eye growth compensates for spectacle lenses. Invest Ophthalmol Vis Sci. 1995;19106:S758.
Schaeffel F, Howland HC, Farkas L. Natural accommodation in the growing chicken. Vis Res. 1986;26:1977–93.
Whatham AR, Judge SJ. Compensatory changes in eye growth and refraction induced by daily wear of soft contact lenses in young marmosets. Vis Res. 2001;41:267–73.
Zhu X, Winawer JA, Wallman J. Potency of myopic defocus in spectacle lens compensation. Invest Ophthalmol Vis Sci. 2003;44:2818–27.
Chiang ST, Phillips JR, Backhouse S. Effect of retinal image defocus on the thickness of the human choroid. Ophthalmic Physiol Opt. 2015;35:405–13.
Read SA, Collins MJ, Sander BP. Human optical axial length and defocus. Invest Ophthalmol Vis Sci. 2010;51:6262–9.
Wang D, Chun RKM, Liu M, et al. Optical defocus rapidly changes choroidal thickness in school children. PLoS One. 2016;11:e0161535.
Chakraborty R, Read SA, Collins MJ. Hyperopic defocus and diurnal changes in human choroid and axial length. Optom Vis Sci. 2013;90:1187–98.
Chakraborty R, Read SA, Collins MJ. Monocular myopic defocus and daily changes in axial length and choroidal thickness of human eyes. Exp Eye Res. 2012;103:47–54.
Moderiano D, Do M, Hobbs S, et al. Influence of the time of day on axial length and choroidal thickness changes to hyperopic and myopic defocus in human eyes. Exp Eye Res. 2019;182:125–36.
Zhu X, Wallman J. Temporal properties of compensation for positive and negative spectacle lenses in chicks. Invest Ophthalmol Vis Sci. 2009;50:37–46.
Zhu X. Temporal integration of visual signals in lens compensation (a review). Exp Eye Res. 2013;114:69–76.
Winawer J, Wallman J. Temporal constraints on lens compensation in chicks. Vis Res. 2002;42:2651–68.
Zhu X, Park TW, Winawer J, Wallman J. In a matter of minutes, the eye can know which way to grow. Invest Ophthalmol Vis Sci. 2005;46:2238–41.
Wallman J, Adams JI. Developmental aspects of experimental myopia in chicks: susceptibility, recovery and relation to emmetropization. Vis Res. 1987;27:1139–63.
Norton TT. Experimental myopia in tree shrews. Ciba Found Symp. 1990;155:178–94.
Nickla DL, Wildsoet C, Wallman J. Visual influences on diurnal rhythms in ocular length and choroidal thickness in chick eyes. Exp Eye Res. 1998;66:163–81.
Troilo D, Wallman J. The regulation of eye growth and refractive state: an experimental study of emmetropization. Vis Res. 1991;31:1237–50.
Schaeffel F, Troilo D, Wallman J, Howland HC. Developing eyes that lack accommodation grow to compensate for imposed defocus. Vis Neurosci. 1990;4:177–83.
Schwahn HN, Schaeffel F. Chick eyes under cycloplegia compensate for spectacle lenses despite six-hydroxy dopamine treatment. Invest Ophthalmol Vis Sci. 1994;35:3516–24.
Chung KM. Critical review: effects of optical defocus on refractive development and ocular growth and relation to accommodation. Optom Vis Sci. 1993;70:228–33.
Morgan IG, Ashby RS, Nickla DL. Form deprivation and lens-induced myopia: are they different? Ophthalmic Physiol Opt. 2013;33:355–61.
C-S K, Marzani D, Wallman J. Differences in time course and visual requirements of ocular responses to lenses and diffusers. Invest Ophthalmol Vis Sci. 2001;42:575–83.
Bartmann M, Schaeffel F, Hagel G, Zrenner E. Constant light affects retinal dopamine levels and blocks deprivation myopia but not lens-induced refractive errors in chickens. Vis Neurosci. 1994;11:199–208.
Padmanabhan V, Shih J, Wildsoet CF. Constant light rearing disrupts compensation to imposed- but not induced-hyperopia and facilitates compensation to imposed myopia in chicks. Vis Res. 2007;47:1855–68.
Smith EL, Hung LF, Huang J. Protective effects of high ambient lighting on the development of form-deprivation myopia in rhesus monkeys. Invest Ophthalmol Vis Sci. 2012;53:421–8.
Ashby RS, Schaeffel F. The effect of bright light on lens compensation in chicks. Invest Ophthalmol Vis Sci. 2010;51:5247–53.
Feldkaemper M, Schaeffel F. An updated view on the role of dopamine in myopia. Exp Eye Res. 2013;114:106–19.
Dong F, Zhi Z, Pan M, et al. Inhibition of experimental myopia by a dopamine agonist: different effectiveness between form deprivation and hyperopic defocus in guinea pigs. Mol Vis. 2011;17:2824–34.
Harper AR, Summers JA. The dynamic sclera: extracellular matrix remodeling in normal ocular growth and myopia development. Exp Eye Res. 2015;133:100–11.
Crewther DP. The role of photoreceptors in the control of refractive state. Prog Retin Eye Res. 2000;19:421–57.
Wildsoet CF. Neural pathways subserving negative lens-induced emmetropization in chicks-insights from selective lesions of the optic nerve and ciliary nerve. Curr Eye Res. 2003;27:371–85.
Wallman J, Gottlieb MD, Rajaram V, Fugate-Wentzek LA. Local retinal regions control local eye growth and myopia. Science. 1987;237:73–7.
Diether S, Schaeffel F. Local changes in eye growth induced by imposed local refractive error despite active accommodation. Vis Res. 1997;37:659–68.
Smith EL, Huang J, Hung LF, Blasdel TL, Humbird TL, Bockhorst KH. Hemiretinal form deprivation: evidence for local control of eye growth and refractive development in infant monkeys. Invest Ophthalmol Vis Sci. 2009;50:5057–69.
Stone RA, Lin T, Laties AM, Iuvone PM. Retinal dopamine and form-deprivation myopia. Proc Natl Acad Sci U S A. 1989;86:704–6.
Iuvone PM, Tigges M, Stone RA, Lambert S, Laties AM. Effects of apomorphine, a dopamine receptor agonist, on ocular refraction and axial elongation in a primate model of myopia. Invest Ophthalmol Vis Sci. 1991;32:1674–7.
Nickla DL, Wilken E, Lytle G, Yom S, Mertz J. Inhibiting the transient choroidal thickening response using the nitric oxide synthase inhibitor l-NAME prevents the ameliorative effects of visual experience on ocular growth in two different visual paradigms. Exp Eye Res. 2006;83:456–64.
Nickla DL, Wildsoet CF. The effect of the nonspecific nitric oxide synthase inhibitor NG-nitro-L-arginine methyl ester on the choroidal compensatory response to myopic defocus in chickens. Optom Vis Sci. 2004;81:111–8.
Feldkaemper MP, Schaeffel F. Evidence for a potential role of glucagon during eye growth regulation in chicks. Vis Neurosci. 2002;19:755–66.
Zhou X, Pardue MT, Iuvone PM, Qu J. Dopamine signaling and myopia development: what are the key challenges. Prog Retin Eye Res. 2017;61:60–71.
Pendrak K, Nguyen T, Lin T, Capehart C, Zhu X, Stone RA. Retinal dopamine in the recovery from experimental myopia. Curr Eye Res. 1997;16:152–7.
Iuvone PM, Tigges M, Fernandes A, Tigges J. Dopamine synthesis and metabolism in rhesus monkey retina: development, aging, and the effects of monocular visual deprivation. Vis Neurosci. 1989;2:465–71.
Crewther DP, Crewther SG, Xie RZ. Changes in eye growth produced by drugs which affect retinal ON or OFF responses to light. J Ocul Pharmacol Ther. 1996;12:193–208.
Crewther DP, Crewther SG. Pharmacological modification of eye growth in normally reared and visually deprived chicks. Curr Eye Res. 1990;9:733–40.
Chakraborty R, Park H, Aung MH, et al. Comparison of refractive development and retinal dopamine in OFF pathway mutant and C57BL/6J wild-type mice. Mol Vis. 2014;20:1318–27.
Chakraborty R, Pardue MT. Molecular and biochemical aspects of the retina on refraction. Prog Mol Biol Transl Sci. 2015;134:249–67.
Chakraborty R, Park HN, Hanif AM, Sidhu CS, Iuvone PM, Pardue MT. ON pathway mutations increase susceptibility to form-deprivation myopia. Exp Eye Res. 2015;137:79–83.
Bergen MA, Chakraborty R, Landis EG, Sidhu C, He L, Iuvone PM, Pardue MT. Altered refractive development in mice with reduced levels of retinal dopamine. Invest Ophthalmol Vis Sci. 2016;57(10):4412–9.
Pardue MT, Faulkner AE, Fernandes A, et al. High susceptibility to experimental myopia in a mouse model with a retinal on pathway defect. Invest Ophthalmol Vis Sci. 2008;49:706–12.
Markand S, Baskin NL, Chakraborty R, et al. IRBP deficiency permits precocious ocular development and myopia. Mol Vis. 2016;22:1291–308.
Zhang Q, Xiao X, Li S, et al. Mutations in NYX of individuals with high myopia, but without night blindness. Mol Vis. 2007;13:330–6.
Xu X, Li S, Xiao X, Wang P, Guo X, Zhang Q. Sequence variations of GRM6 in patients with high myopia. Mol Vis. 2009;15:2094–100.
Pras E, Abu A, Rotenstreich Y, et al. Cone-rod dystrophy and a frameshift mutation in the PROM1 gene. Mol Vis. 2009;15:1709.
Sieving PA, Fishman GA. Refractive errors of retinitis pigmentosa patients. Br J Ophthalmol. 1978;62:163–7.
Charman WN. Aberrations and myopia. Ophthalmic Physiol Opt. 2005;25:285–301.
Wildsoet CF, Atchison DA, Collins MJ. Longitudinal chromatic aberration as a function of refractive error. Clin Exp Optom. 1993;76:119–22.
Little JA, McCullough SJ, Breslin KM, Saunders KJ. Higher order ocular aberrations and their relation to refractive error and ocular biometry in children. Invest Ophthalmol Vis Sci. 2014;55:4791–800.
Buehren T, Collins MJ, Carney LG. Near work induced wavefront aberrations in myopia. Vis Res. 2005;45:1297–312.
Vincent SJ, Collins MJ, Read SA, Carney LG, Yap MK. Corneal changes following near work in myopic anisometropia. Ophthalmic Physiol Opt. 2013;33:15–25.
Hiraoka T, Kotsuka J, Kakita T, Okamoto F, Oshika T. Relationship between higher-order wavefront aberrations and natural progression of myopia in schoolchildren. Sci Rep. 2017;7:7876.
Atchison DA, Li SM, Li H, et al. Relative peripheral hyperopia does not predict development and progression of myopia in children. Invest Ophthalmol Vis Sci. 2015;56:6162–70.
Lee TT, Cho P. Relative peripheral refraction in children: twelve-month changes in eyes with different ametropias. Ophthalmic Physiol Opt. 2013;33:283–93.
Cheng X, Xu J, Chehab K, Exford J, Brennan N. Soft contact lenses with positive spherical aberration for myopia control. Optom Vis Sci. 2016;93:353–66.
Hiraoka T, Okamoto C, Ishii Y, Kakita T, Oshika T. Contrast sensitivity function and ocular higher-order aberrations following overnight orthokeratology. Invest Ophthalmol Vis Sci. 2007;48:550–6.
Hiraoka T, Miyata K, Nakamura Y, et al. Influences of cycloplegia with topical atropine on ocular higher-order aberrations. Ophthalmology. 2013;120:8–13.
Huang HM, Chang DS, Wu PC. The association between near work activities and myopia in children-a systematic review and meta-analysis. PLoS One. 2015;10:e0140419.
Rosenfield M, Gilmartin B. Disparity-induced accommodation in late-onset myopia. Ophthalmic Physiol Opt. 1988;8:353–5.
Gwiazda J, Thorn F, Bauer J, Held R. Myopic children show insufficient accommodative response to blur. Invest Ophthalmol Vis Sci. 1993;34:690–4.
McBrien NA, Millodot M. The effect of refractive error on the accommodative response gradient. Ophthalmic Physiol Opt. 1986;6:145–9.
Gwiazda J, Bauer J, Thorn F, Held R. A dynamic relationship between myopia and blur-driven accommodation in school-aged children. Vis Res. 1995;35:1299–304.
Abbott ML, Schmid KL, Strang NC. Differences in the accommodation stimulus response curves of adult myopes and emmetropes. Ophthalmic Physiol Opt. 1998;18:13–20.
Cheng D, Schmid KL, Woo GC, Drobe B. Randomized trial of effect of bifocal and prismatic bifocal spectacles on myopic progression: two-year results. Arch Ophthalmol. 2010;128:12–9.
Berntsen DA, Barr CD, Mutti DO, Zadnik K. Peripheral defocus and myopia progression in myopic children randomly assigned to wear single vision and progressive addition lenses. Invest Ophthalmol Vis Sci. 2013;54:5761–70.
Mutti DO, Mitchell GL, Jones LA, et al. Accommodation, acuity, and their relationship to emmetropization in infants. Optom Vis Sci. 2009;86:666–76.
Weiss S, Schaeffel F. Diurnal growth rhythms in the chicken eye: relation to myopia development and retinal dopamine levels. J Comp Physiol A. 1993;172:263–70.
Chakraborty R, Read SA, Collins MJ. Diurnal variations in axial length, choroidal thickness, intraocular pressure, and ocular biometrics. Invest Ophthalmol Vis Sci. 2011;52:5121–9.
Brown JS, Flitcroft DI, Ying GS, et al. In vivo human choroidal thickness measurements: evidence for diurnal fluctuations. Invest Ophthalmol Vis Sci. 2009;50:5–12.
Stone RA, Quinn GE, Francis EL, et al. Diurnal axial length fluctuations in human eyes. Invest Ophthalmol Vis Sci. 2004;45:63–70.
Li T, Troilo D, Glasser A, Howland HC. Constant light produces severe corneal flattening and hyperopia in chickens. Vis Res. 1995;35:1203–9.
Guyton DL, Greene PR, Scholz RT. Dark-rearing interference with emmetropization in the rhesus monkey. Invest Ophthalmol Vis Sci. 1989;30:761–4.
Nickla DL, Totonelly K. Brief light exposure at night disrupts the circadian rhythms in eye growth and choroidal thickness in chicks. Exp Eye Res. 2016;146:189–95.
Nickla DL. The phase relationships between the diurnal rhythms in axial length and choroidal thickness and the association with ocular growth rate in chicks. J Comp Physiol A. 2006;192:399–407.
Kearney S, O’Donoghue L, Pourshahidi LK, Cobice D, Saunders KJ. Myopes have significantly higher serum melatonin concentrations than non-myopes. Ophthalmic Physiol Opt. 2017;37:557–67.
Jee D, Morgan IG, Kim EC. Inverse relationship between sleep duration and myopia. Acta Ophthalmol. 2016;94:e204–10.
Mutti DO, Mitchell GL, Moeschberger ML, Jones LA, Zadnik K. Parental myopia, near work, school achievement, and children’s refractive error. Invest Ophthalmol Vis Sci. 2002;43:3633–40.
Morgan IG. The biological basis of myopic refractive error. Clin Exp Optom. 2003;86:276–88.
Au Eong KG, Tay TH, Lim MK. Education and myopia in 110,236 young Singaporean males. Singap Med J. 1993;34:489–92.
Williams KM, Bertelsen G, Cumberland P, et al. Increasing prevalence of myopia in Europe and the impact of education. Ophthalmology. 2015;122:1489–97.
Mirshahi A, Ponto KA, Hoehn R, et al. Myopia and level of education: results from the Gutenberg Health Study. Ophthalmology. 2014;121:2047–52.
Read SA, Collins MJ, Woodman EC, Cheong SH. Axial length changes during accommodation in myopes and emmetropes. Optom Vis Sci. 2010;87:656–62.
Woodman-Pieterse EC, Read SA, Collins MJ, Alonso-Caneiro D. Regional changes in choroidal thickness associated with accommodation. Invest Ophthalmol Vis Sci. 2015;56:6414–22.
Hepsen IF, Evereklioglu C, Bayramlar H. The effect of reading and near-work on the development of myopia in emmetropic boys: a prospective, controlled, three-year follow-up study. Vis Res. 2001;41:2511–20.
Jones LA, Sinnott LT, Mutti DO, Mitchell GL, Moeschberger ML, Zadnik K. Parental history of myopia, sports and outdoor activities, and future myopia. Invest Ophthalmol Vis Sci. 2007;48:3524–32.
He M, Zheng Y, Xiang F. Prevalence of myopia in urban and rural children in mainland China. Optom Vis Sci. 2009;86:40–4.
He M, Huang W, Zheng Y, Huang L, Ellwein LB. Refractive error and visual impairment in school children in rural southern China. Ophthalmology. 2007;114:374–82.
Zhang M, Li L, Chen L, et al. Population density and refractive error among Chinese children. Invest Ophthalmol Vis Sci. 2010;51:4969–76.
Ip JM, Rose KA, Morgan IG, Burlutsky G, Mitchell P. Myopia and the urban environment: findings in a sample of 12-year-old Australian school children. Invest Ophthalmol Vis Sci. 2008;49:3858–63.
Choi KY, Yu WY, Lam CHI, et al. Childhood exposure to constricted living space: a possible environmental threat for myopia development. Ophthalmic Physiol Opt. 2017;37:568–75.
Parssinen O, Lyyra AL. Myopia and myopic progression among schoolchildren: a three-year follow-up study. Invest Ophthalmol Vis Sci. 1993;34:2794–802.
Rose KA, Morgan IG, Ip J, et al. Outdoor activity reduces the prevalence of myopia in children. Ophthalmology. 2008;115:1279–85.
Guo Y, Liu LJ, Tang P, et al. Outdoor activity and myopia progression in 4-year follow-up of Chinese primary school children: The Beijing Children Eye Study. PLoS One. 2017;12:e0175921.
Guggenheim JA, Northstone K, McMahon G, et al. Time outdoors and physical activity as predictors of incident myopia in childhood: a prospective cohort study. Invest Ophthalmol Vis Sci. 2012;53:2856–65.
Parssinen O, Kauppinen M, Viljanen A. The progression of myopia from its onset at age 8-12 to adulthood and the influence of heredity and external factors on myopic progression. A 23-year follow-up study. Acta Ophthalmol. 2014;92:730–9.
Jones-Jordan LA, Sinnott LT, Cotter SA, et al. Time outdoors, visual activity, and myopia progression in juvenile-onset myopes. Invest Ophthalmol Vis Sci. 2012;53:7169–75.
Sherwin JC, Reacher MH, Keogh RH, Khawaja AP, Mackey DA, Foster PJ. The association between time spent outdoors and myopia in children and adolescents: a systematic review and meta-analysis. Ophthalmology. 2012;119:2141–51.
Flitcroft DI. The complex interactions of retinal, optical and environmental factors in myopia aetiology. Prog Retin Eye Res. 2012;31:622–60.
Cohen Y, Belkin M, Yehezkel O, Solomon AS, Polat U. Dependency between light intensity and refractive development under light-dark cycles. Exp Eye Res. 2011;92:40–6.
Karouta C, Ashby RS. Correlation between light levels and the development of deprivation myopia. Invest Ophthalmol Vis Sci. 2014;56:299–309.
Smith EL, Hung LF, Arumugam B, Huang J. Negative lens-induced myopia in infant monkeys: effects of high ambient lighting. Invest Ophthalmol Vis Sci. 2013;54:2959–69.
Read SA, Collins MJ, Vincent SJ. Light exposure and eye growth in childhood. Invest Ophthalmol Vis Sci. 2015;56:6779–87.
Ostrin LA, Sajjadi A, Benoit JS. Objectively measured light exposure during school and summer in children. Optom Vis Sci. 2018;95:332–42.
Wu PC, Chen CT, Lin KK, et al. Myopia prevention and outdoor light intensity in a school-based cluster randomized trial. Ophthalmology. 2018;125(8):1239–50.
Rudnicka AR, Kapetanakis VV, Wathern AK, et al. Global variations and time trends in the prevalence of childhood myopia, a systematic review and quantitative meta-analysis: implications for aetiology and early prevention. Br J Ophthalmol. 2016;100:882–90.
French AN, Morgan IG, Burlutsky G, Mitchell P, Rose KA. Prevalence and 5- to 6-year incidence and progression of myopia and hyperopia in Australian school children. Ophthalmology. 2013;120:1482–91.
Read SA, Vincent SJ, Tan CS, Ngo C, Collins MJ, Saw SM. Patterns of daily outdoor light exposure in Australian and Singaporean children. Transl Vis Sci Technol. 2018;7:8.
Lan W, Feldkaemper M, Schaeffel F. Intermittent episodes of bright light suppress myopia in the chicken more than continuous bright light. PLoS One. 2014;9:e110906.
He M, Xiang F, Zeng Y, et al. Effect of time spent outdoors at school on the development of myopia among children in China: a randomized clinical trial. JAMA. 2015;314:1142–8.
Wu PC, Tsai CL, Wu HL, Yang YH, Kuo HK. Outdoor activity during class recess reduces myopia onset and progression in school children. Ophthalmology. 2013;120:1080–5.
Torii H, Kurihara T, Seko Y, et al. Violet light exposure can be a preventive strategy against myopia progression. EBioMedicine. 2017;15:210–9.
Foulds WS, Barathi VA, Luu CD. Progressive myopia or hyperopia can be induced in chicks and reversed by manipulation of the chromaticity of ambient light. Invest Ophthalmol Vis Sci. 2013;54:8004–12.
Smith EL, Hung LF, Arumugam B, Holden BA, Neitz M, Neitz J. Effects of long-wavelength lighting on refractive development in infant rhesus monkeys. Invest Ophthalmol Vis Sci. 2015;56:6490–500.
Gawne TJ, Ward AH, Norton TT. Long-wavelength (red) light produces hyperopia in juvenile and adolescent tree shrews. Vis Res. 2017;140:55–65.
Jiang L, Zhang S, Schaeffel F, et al. Interactions of chromatic and lens-induced defocus during visual control of eye growth in guinea pigs (Cavia porcellus). Vis Res. 2014;94:24–32.
Download references
Author information
Authors and affiliations.
Flinders University, Adelaide, SA, Australia
Ranjay Chakraborty
Queensland University of Technology, Brisbane, QLD, Australia
Scott A. Read & Stephen J. Vincent
You can also search for this author in PubMed Google Scholar
Corresponding author
Correspondence to Ranjay Chakraborty .
Editor information
Editors and affiliations.
Singapore National Eye Center, Duke-NUS Medical School, National University of Singapore, Singapore
Tien Y. Wong
Rights and permissions
Open Access This chapter is licensed under the terms of the Creative Commons Attribution 4.0 International License (http://creativecommons.org/licenses/by/4.0/), which permits use, sharing, adaptation, distribution and reproduction in any medium or format, as long as you give appropriate credit to the original author(s) and the source, provide a link to the Creative Commons license and indicate if changes were made.
The images or other third party material in this chapter are included in the chapter's Creative Commons license, unless indicated otherwise in a credit line to the material. If material is not included in the chapter's Creative Commons license and your intended use is not permitted by statutory regulation or exceeds the permitted use, you will need to obtain permission directly from the copyright holder.
Reprints and permissions
Copyright information
© 2020 The Author(s)
About this chapter
Chakraborty, R., Read, S.A., Vincent, S.J. (2020). Understanding Myopia: Pathogenesis and Mechanisms. In: Ang, M., Wong, T. (eds) Updates on Myopia. Springer, Singapore. https://doi.org/10.1007/978-981-13-8491-2_4
Download citation
DOI : https://doi.org/10.1007/978-981-13-8491-2_4
Published : 10 October 2019
Publisher Name : Springer, Singapore
Print ISBN : 978-981-13-8490-5
Online ISBN : 978-981-13-8491-2
eBook Packages : Medicine Medicine (R0)
Share this chapter
Anyone you share the following link with will be able to read this content:
Sorry, a shareable link is not currently available for this article.
Provided by the Springer Nature SharedIt content-sharing initiative
- Publish with us
Policies and ethics
- Find a journal
- Track your research
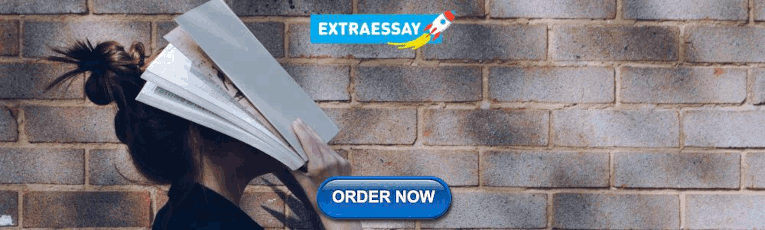
IMAGES
VIDEO
COMMENTS
Prevalence. Its prevalence rate in the last few years has increased and varied through regions and races in various parts of the world. Peoples in developed countries are more myopic due to strong environmental and competitive educational systems.[14,15] Increased risk factors for myopia have been identified as more time spent on near-sighted work (computer, cell phone, video game), time spent ...
A largely unrecognized epidemic of nearsightedness, or myopia, is afflicting the eyes of children. People with myopia can see close-up objects clearly, like the words on a page. But their distance ...
Abstract. Myopia poses a significant burden on the healthcare system, economy and quality of life. It is an emerging global public health challenge and requires interventions to delay or stop onset and progression. With changing times and evidence, the concepts of myopia are changing along with the treatment and control strategies.
In 2016, Holden et al. estimated that the global prevalence of myopia was 1.406 billion people worldwide (22.9% of the global population), and that 163 million people had high myopia in 2000. They also concluded that, by 2050, there will be 4.758 billion people with myopia (49.8% of the global population), and 938 million will have high myopia ...
Estimates for Singapore suggest an extremely high prevalence of myopia by 2050, with 83% of the population (5 million of 6 million) having some form of myopia, 15% of whom (900,000 individuals ...
The mechanism linking education to myopia may be defocus signals in the central and peripheral retina 6,18,39-41 and persistent lags of accommodation, 22,25-28 which may stimulate axial elongation. A recent alternative hypothesis suggests that the problem may be associated with the use of black text on a white background, which heavily ...
Published: April 24, 2024 8:37am EDT. Myopia, or the need for corrected vision to focus or see objects at a distance, has become a lot more common in recent decades. Some even consider myopia ...
Smart device exposure might be associated with an increased risk of myopia. Research with objective measures of screen time and myopia-related outcomes that investigates smart device exposure as an independent risk factor is required. ... We searched MEDLINE on May 19, 2020 using natural language search terms, including "smartphone ...
As a result, prevention of the onset of myopia and control of its progression are a high priority. Economic analysis has shown that the epidemic of myopia in young people, and an increasing number ...
Instead of focusing images on the retina—the light-sensitive tissue in the back of the eye—images are focused at a point in front of the retina. As a result, people with myopia have good near vision but poor distance vision.About 41.6 percent of Americans are nearsighted, up from 25 percent in 1971. 1 "The trend appears to be upwards in ...
Abstract. In myopia, the eye grows too long, and the image projected on the retina is poorly focused when subjects look at a distance. While the retina normally controls eye growth by visual ...
Distribution of Journals. Based on the retrieved results, the articles on myopia research were distributed among 164 journals. The top 10 journals that published articles on this topic are listed in Table 3.According to the citations, Investigative Ophthalmology & Visual Science and Ophthalmology ranked first and second, respectively. The Journal of Cataract and Refractive Surgery published ...
Mounting evidence suggests that the cause of myopia can be attributed to the complex interaction of environmental exposure and genetic susceptibility. An increasing number of researchers have focused on the genetic pathogenesis of myopia in recent years. ... HIF-1 α has become a new target of myopia research in recent years, and it may be ...
Kathryn Sill is a web and communications specialist for the Byers Eye Institute in the Department of Ophthalmology, at Stanford University School of Medicine. Email her at [email protected]. The prevalence of myopia, or nearsightedness, continues to grow, affecting about 42% of the entire American population.
INTRODUCTION. Myopia, or nearsightedness, is the most common human eye disorder in the world, and is a significant global public health concern. (1-2) Along with cataract, macular degeneration, infectious disease, and vitamin A deficiency, myopia is one of the most important causes of visual impairment worldwide.(2-3) Severe or high-grade myopia is a leading cause of blindness because of ...
A new study published in one of the world's leading medical journals has revealed a link between screen time and higher risk and severity of myopia, or short-sightedness, in children and young ...
Abstract. The myopia epidemic has become a global public health problem. Although myopia is progressing worldwide, the recent coronavirus infections 2019 (COVID-19) outbreak has spurred myopia progression. The current evidence-based treatments for humans are atropine eye drops, optical treatment with defocus, use of orthokeratology, extending ...
A new study from The University of Western Australia has found the world is experiencing a myopia (short-sightedness) epidemic, and the main cause is computer screens rather than mobile phones or ...
Myopia is a common disorder that occurs in childhood and early adulthood and has become a major cause of blindness. Myopia is defined as parallel light passing through the refractive system of the eye and focusing in front of the retina when the eye is in its relaxed state, which leads to the formation of blurred images [].Myopia is mainly related to axial growth (axial myopia), but a small ...
Charman and Radhakrishnan 150 point out that the relative peripheral hyperopia that is associated with the onset of myopia may be a consequence rather than a cause of the development of myopia. They 151 also draw attention to a report, which suggests that eye shape in people of Chinese origin is more axially symmetric than in Caucasians.
Abstract: Myopia occurs in more than 50% of the population in many industrialized countries and is expected to increase; complications associated with axial elongation from myopia are the sixth leading cause of blindness. Thus, understanding its etiology, epidemiology, and the results of various treatment regiments may modify current care and ...
Recent evidence suggests that the human visual system may also be able to detect the sign and magnitude of imposed defocus and make compensatory changes in axial length, similar to other animals. A number of studies have reported small bidirectional changes in axial length and choroidal thickness in response to 1-2 h of myopic and hyperopic ...
Recent research suggests myopia may be caused by. too much time indoors. The optic disc is the. area of the retina through which the axons of the ganglion cells exit. In _____ cells, light falling in the center of the cell causes excitation, and light falling in the surround causes inhibition.
Smenz228. Preview. quizlette5199889. Preview. Study with Quizlet and memorize flashcards containing terms like recent research suggests myopia may be caused by, which statement about photopic and scotopix vision is false?, the process by which the shape of the lens if altered in order to project a sharp image onto the retina is called and more.