- Open access
- Published: 20 December 2020
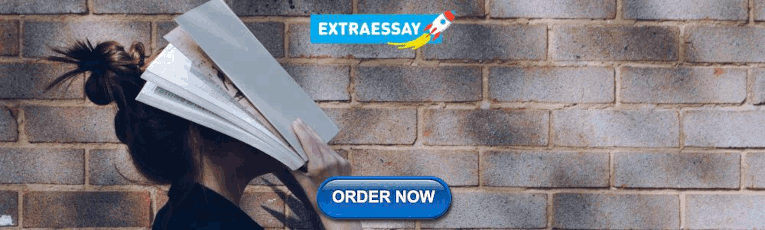
Coronavirus vaccine development: from SARS and MERS to COVID-19
- Yen-Der Li 1 na1 ,
- Wei-Yu Chi 2 na1 ,
- Jun-Han Su 1 ,
- Louise Ferrall 2 ,
- Chien-Fu Hung 2 &
- T.-C. Wu ORCID: orcid.org/0000-0003-1623-4584 2 , 3
Journal of Biomedical Science volume 27 , Article number: 104 ( 2020 ) Cite this article
313k Accesses
258 Citations
1083 Altmetric
Metrics details
Severe Acute Respiratory Syndrome Coronavirus 2 (SARS-CoV-2) is a new type of coronavirus that causes the Coronavirus Disease 2019 (COVID-19), which has been the most challenging pandemic in this century. Considering its high mortality and rapid spread, an effective vaccine is urgently needed to control this pandemic. As a result, the academia, industry, and government sectors are working tightly together to develop and test a variety of vaccines at an unprecedented pace. In this review, we outline the essential coronavirus biological characteristics that are important for vaccine design. In addition, we summarize key takeaways from previous vaccination studies of Severe Acute Respiratory Syndrome Coronavirus (SARS-CoV) and Middle East Respiratory Syndrome Coronavirus (MERS-CoV), highlighting the pros and cons of each immunization strategy. Finally, based on these prior vaccination experiences, we discuss recent progress and potential challenges of COVID-19 vaccine development.
Introduction
Coronaviruses (CoVs) are a group of related viruses that can cause respiratory tract infection in humans ranging from mild symptoms to lethal outcomes. Until now, there are seven genera of CoVs that are known to infect humans [ 1 ]. Four of these genera, including Human Coronavirus 229E (HCoV-229E), Human Coronavirus OC43 (HCoV-OC43), Human Coronavirus NL63 (HCoV-NL63), and Human Coronavirus HKU1 (HCoV-HKU1), only cause relatively mild and self-limiting respiratory symptoms [ 2 ]. Alternatively, the other three CoVs, Severe Acute Respiratory Syndrome Coronavirus (SARS-CoV), Middle East Respiratory Syndrome Coronavirus (MERS-CoV), and Severe Acute Respiratory Syndrome Coronavirus 2 (SARS-CoV-2), are highly pathogenic and can lead to severe respiratory diseases and fatal outcome in infected patients. The first lethal coronavirus SARS-CoV emerged in 2002 in Guangdong Province, China. During the 2002–2004 outbreak, SARS-CoV had infected 8,098 people and resulted in 774 SARS-associated deaths (~ 10% mortality rate) across 29 countries before it disappeared [ 3 ]. In 2012, MERS-CoV emerged in Saudi Arabia. It caused two outbreaks in South Korea in 2015 and in Saudi Arabia in 2018, and still has ongoing reports of sporadic cases nowadays. As of January 2020, there are 2,519 confirmed MERS cases and 866 deaths (~ 35% mortality rate) across 27 countries [ 4 ]. In December 2019, a new type of CoV that can cause severe respiratory illness emerged in Wuhan, China. The World Health Organization named this novel virus SARS-CoV-2 and the disease COVID-19, or Coronavirus Disease 2019. The clinical manifestation of COVID-19 can vary from asymptomatic and mild flu-like symptoms to acute respiratory distress syndrome and death. Long-term pulmonary, cardiological, and neurological complications have also been reported in COVID-19 cases [ 5 ]. Compared with SARS-CoV and MERS-CoV, SARS-CoV-2 is highly contagious with an estimated reproductive number of 2.2 (one existing COVID-19 case can cause an average of 2.2 new infections) [ 6 ]. In addition, its ability to spread through asymptomatic patients has posed a great challenge to containment measures [ 7 ]. By October 2020, SARS-CoV-2 has infected more than 43 million individuals and resulted in about 1.15 million deaths (~ 3% mortality rate) in 235 countries, areas or territories worldwide [ 8 ]. Needless to say, COVID-19 has become the most serious public health crisis of our generation and has a profound impact on the global economy and geopolitics. Although our understanding of pathogenic CoVs has been steadily accumulating for about two decades, no effective vaccine has yet been approved for the prevention of human CoV infection. Considering the rapid spread and high mortality of COVID-19, an effective vaccine is urgently needed to control this pandemic. In this review, we summarize relevant CoV biology, SARS and MERS immunization strategies, and recent efforts of COVID-19 vaccine development. We hope this review can provide essential knowledge for any researcher who is interested in COVID-19 vaccine development.
Coronavirus biology and its implication on vaccine development
Coronaviruses, whose name derives from their characteristic crown-like appearance under the electron microscope, are enveloped RNA viruses with a diameter of approximately 80–160 nm [ 9 , 10 ]. The genome of CoVs is a ~ 30 kb single-stranded positive-sense RNA molecule, which is the largest genome of all known RNA viruses [ 9 , 10 , 11 ]. The 5′-terminus of the CoV genome contains two overlapping open reading frames (ORFs): ORF 1a and ORF 1b, spanning two-thirds of the genome length (Fig. 1 a) [ 9 , 10 , 11 ]. ORF 1a and ORF 1ab can be translated into two polyproteins (pp), pp1a and pp1ab, which are further cleaved into 16 non-structural proteins (Nsps) involved in viral genome replication and subgenomic mRNA synthesis [ 9 , 10 , 11 ]. The 3′-terminus of the CoV genome encodes four major structural proteins in the order of spike (S), envelope (E), membrane (M), and nucleocapsid (N) proteins (Fig. 1 a) [ 9 , 10 , 11 ]. S, E, M protein forms the envelope of the CoV, while N protein forms the capsid to pack genomic RNA (Fig. 1 b) [ 9 , 10 , 11 ]. The 3′-terminus of the genome also encodes multiple accessory proteins, which are usually genus-specific and can help CoV evade the immune system or increase virulence [ 9 , 10 , 11 ]. For instance, SARS-CoV contains accessory protein ORF 3a, 3b, 6, 7a, 7b, 8a, 8b and 9b, MERS-CoV contains ORF 3, 4a, 4b, 5, 8b, and SARS-CoV-2 contains ORF 3a, 6, 7a, 7b, 8, 10 (Fig. 1 a) [ 12 , 13 , 14 ].
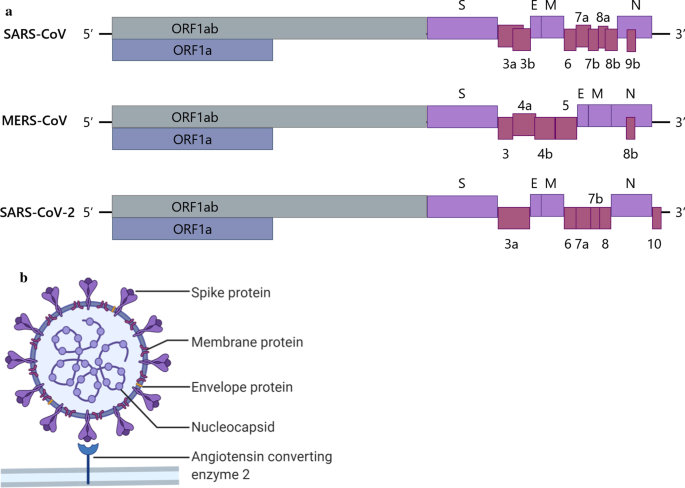
The genome and virion structure of coronaviruses (CoVs). a The genome structure of SARS-CoV, MERS-CoV, and SARS-CoV-2 [ 12 , 13 , 14 ]. The 5′-terminus of the CoV genome contains two overlapping open reading frames (ORFs): ORF 1a and ORF 1b, spanning two-thirds of the genome length. ORF 1a and ORF 1ab can be translated into two polyproteins (pp), pp1a and pp1ab, which are further cleaved into 16 non-structural proteins (Nsps). The 3′-terminus of the CoV genome encodes four major structural proteins in the order of spike (S), envelope (E), membrane (M), and nucleocapsid (N) proteins. Genus-specific accessory proteins are also encoded at the 3′-terminus of the CoV genome. b The virion structure of SARS-CoV-2 [ 16 ]. The spike (S), envelope (E), membrane (M) proteins form the envelope of the CoV, and the nucleocapsid (N) proteins form the capsid to pack the genomic RNA. The spike protein binds to angiotensin converting enzyme 2 (ACE2) on the cell membrane, which allows the virus to enter the cell. (Created with BioRender.com.)
Many viral proteins are essential for the life cycle of CoVs. For entering target cells, S protein first binds to cellular receptors through its receptor-binding domain (RBD), and the receptor-virus complex is subsequently translocated to endosomes (Fig. 2 ) [ 15 ]. Both SARS-CoV and SARS-CoV-2 S proteins bind to angiotensin-converting enzyme 2 (ACE2), while the S protein of MERS-CoV uses dipeptidyl peptidase-4 (DPP4) as its cellular receptor (Fig. 1 b) [ 16 ]. At the endosome, S protein is further cleaved into S1 (RBD-containing) and S2 (non-RBD-containing) subunits, and the S2 subunit mediates fusion between the viral envelope and the host cell membrane [ 15 ]. After entering the cell, several Nsps, particularly RNA‐dependent RNA polymerase (Nsp12) and helicase (Nsp13), mediate the replication of the CoV genome and the transcription of CoV mRNA [ 17 ]. The CoV mRNA is further translated into different nonstructural and structural proteins. The N proteins bind to CoV genomic RNA to form viral nucleocapsids, and S, E, M proteins form the envelope of CoV [ 15 ]. After assembly, viral particles bud through an endoplasmic reticulum (ER)-Golgi pathway and exit the cells by exocytosis (Fig. 2 ) [ 15 ].
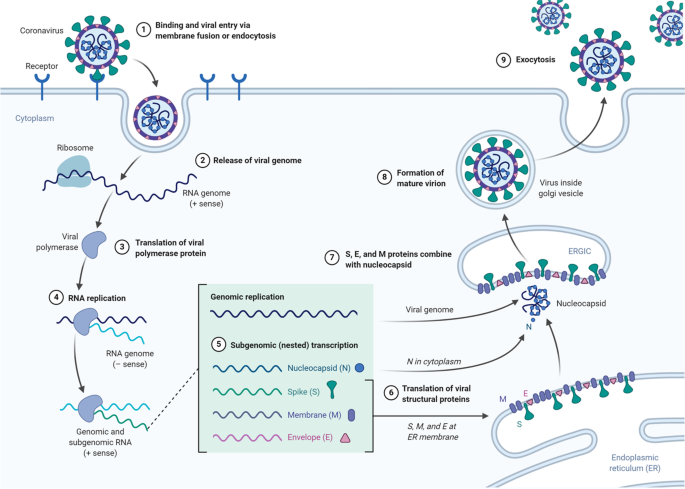
The life cycle of SARS-CoV-2 [ 9 , 10 , 15 ]. Upon binding to the membrane receptor ACE2, SARS-CoV-2 virion enters the host cell and releases its plus-strand RNA genome. The plus-strand RNA translates pp1a and pp1ab, which are further cleaved into multiple non-structural proteins (Nsps) including an RNA-dependent RNA polymerase (Nsp12). The RNA-dependent RNA polymerase transcribes a negative-strand genomic RNA, and then uses this negative-strand genomic RNA as template to generate more plus-strand genomic RNA (genomic replication) and many different subgenomic RNAs (subgenomic transcription). The subgenomic RNAs are further translated into major structural proteins (N, S, M, E), which will assemble with plus-strand genomic RNA to form a mature virion in lumen of the ER. Finally, the whole virus leaves the cell through exocytosis. (Reprinted from “Coronavirus Replication Cycle”, by BioRender.com (2020). Retrieved from https://app.biorender.com/biorender-templates )
The S protein is particularly important for virus-cell receptor binding and virus-cell membrane fusion, suggesting that it can be an effective target for CoV vaccine design [ 15 ]. In fact, studies have shown that antibodies generated against the S protein are long-lasting and immunodominant in recovered SARS patients [ 18 , 19 ]. In addition, several studies have demonstrated that the anti-S antibody can neutralize SARS-CoV and MERS-CoV and provides protective effects in animals and humans [ 20 , 21 , 22 ]. Moreover, many S protein-based vaccines against SARS-CoV and MERS-CoV have been shown to elicit potent immune responses and protective effects in preclinical models [ 23 , 24 , 25 , 26 , 27 ]. These results corroborate that CoV S protein serves as an ideal vaccine target to induce neutralizing antibodies and protective immunity. Besides S protein, other structural proteins have also been tested as vaccine targets. N protein-based vaccines usually cannot induce neutralizing antibodies, likely due to the fact that N protein is not displayed on the CoV surface [ 16 ]. However, N protein has the advantage of being more conserved across CoV species than S protein, making it a potential target for a T-cell inducing, universal CoV vaccine [ 16 ]. One recent study has shown that a viral vector vaccine expressing N protein can induce CD4+ T cell-dependent protection against SARS-CoV and MERS-CoV, suggesting the feasibility of N protein-based T-cell inducing CoV vaccines [ 28 ]. M protein-based vaccines, on the other hand, can induce a high titer of antibody response in immunized animals [ 29 ]. However, no neutralization antibody or protective immunity data of M protein-based vaccines in preclinical models have been demonstrated. Finally, very few CoV E protein-based immunization studies have been reported so far, and none of the studies demonstrated induction of neutralizing antibodies or protective immunity [ 30 ].
There are also immunopathological complications associated with the SARS-CoV and MERS-CoV vaccines that require addressing and further optimization. One adverse effect is the induction of antibody-dependent enhancement (ADE) effect, which is usually caused by vaccine-induced suboptimal antibodies that facilitates viral entry into host cells [ 11 , 31 ]. A study found that SARS-CoV vaccine based on full-length S protein enhances SARS-CoV infection of human cell lines in vitro [ 32 ]. Additionally, two studies have also shown that anti-S protein serum results in increased viral infectivity of SARS-CoV [ 33 , 34 ]. These results raise safety concerns for S protein-based SARS-CoV and MERS-CoV vaccines. One potential strategy to overcome the ADE problem is to design vaccines that only contain major neutralizing epitopes, such as the S1 subunit or the RBD domain of the S protein. This strategy can decrease the induction of non-neutralizing antibodies by CoV vaccines and therefore reduce the ADE effect. Another potential adverse effect is vaccine-induced eosinophilic immunopathology, which is an unwanted Th2-skewed immune response elicited by vaccination [ 11 , 35 ]. At least two studies have reported that whole inactivated virus vaccine of SARS-CoV induces eosinophilic proinflammatory pulmonary response after mice challenged with SARS-CoV [ 36 , 37 ]. In addition, one study also reported that immunization with SARS-CoV virus-like particle (VLP) vaccine leads to eosinophilic immunopathology in the lung after viral challenge [ 37 ]. In order to prevent this Th2-type immunopathology, a few studies have worked on adjuvant optimization. They found that appropriate adjuvants, such as Toll-like receptor agonist and delta-inulin polysaccharide, can increase serum neutralizing antibody titers and reduce lung eosinophilic immunopathology [ 38 , 39 ]. Their results provide a promising strategy to deal with Th2-skewed immune response induced by some CoV vaccines.
Previous progress of SARS-CoV and MERS-CoV immunization strategies
Various forms of vaccines targeting SARS-CoV and MERS-CoV have been developed and tested in preclinical models. However, only a few of them entered clinical trials and none of them have been FDA approved. These approaches include protein subunit vaccines, virus-like particle vaccines, DNA vaccines, viral vector vaccines, whole-inactivated vaccines and live-attenuated vaccines. The following sections outline the principles of various forms of SARS-CoV and MERS-CoV vaccine development (Table 1 ), and the latest results from both preclinical studies and clinical trials (Table 2 ).
Protein subunit vaccine
Protein subunit vaccines consist of viral antigenic fragments produced by recombinant protein techniques. They are easy to produce, and relatively safe and well-tolerated compared to whole virus vaccines and viral vector vaccines. The drawback of protein subunit vaccines is their low immunogenicity. Therefore, adjuvants and fusion with immunostimulatory molecules are usually used together with subunit vaccines to overcome this challenge.
The development of SARS-CoV protein subunit vaccines was initially surrounding full-length S protein-based vaccines and then later focused on S protein RBD-based vaccines. None of the SARS-CoV protein subunit vaccines entered clinical trials, but they induced potent antibody responses and protective effects in preclinical models [ 23 , 24 , 32 , 40 , 41 , 42 , 43 , 44 ]. Studies have shown that full-length S protein, extracellular domain of the S protein, and trimeric S proteins (triSpike) are all immunogenic and can elicit protection against SARS-CoV infection [ 23 , 24 , 32 ]. However, Kam et al. and Jamue et al. have found that triSpike vaccine can also cause Fcγ receptor II (FcγRII)-dependent SARS-CoV infection in human B cells in vitro [ 32 , 33 ]. On the other hand, S protein RBD-based vaccines are able to induce high-titer neutralizing antibodies without causing obvious pathogenic effects [ 40 , 41 , 42 , 43 , 44 ]. This is probably because RBD-based vaccines do not contain additional non-neutralizing epitopes as full-length S protein vaccines do. One study has shown that RBD-based vaccines not only protect most of the SARS-CoV challenged mice with no detectable viral RNA in the lung, but can also induce long-lasting S-specific antibodies that can be maintained for 12 months [ 42 ]. Furthermore, RBD-based SARS-CoV vaccines have also been shown to induce RBD-specific IFN-γ producing cellular immune responses in mice [ 44 ]. As a result, SARS-CoV RBD has become the main target for SARS vaccines. Finally, SARS-CoV subunit vaccines based on S2 subunit, N and M structural proteins have also been tested [ 29 , 45 , 46 ]. However, no evidence has shown that they can induce neutralizing antibodies or protective effects against viral challenge.
Guided by previous SARS-CoV experiences, most proteins subunit vaccines of MERS-CoV are focused on RBD-based vaccines. RBD-based MERS-CoV vaccines generally show great immunogenicity and elicit potent neutralizing antibodies, cell-mediated immunity, and protective effect against MERS-CoV infection [ 25 , 26 ]. One study from Tai et al. found that trimeric RBD protein vaccines can induce long-lasting neutralizing antibodies for 6 months [ 26 ]. Another study also from Tai et al. demonstrated that recombinant RBD proteins from different MERS-CoV strains can induce antibodies that cross-neutralize with divergent human and camel MERS-CoV strains [ 25 ]. These results indicate that MERS-CoV RBD serves as a promising vaccine target with the ability to induce long-lasting and broad-spectrum neutralizing antibodies against infection. Other than RBD-based vaccines, RBD-containing S1 subunit vaccines have also been shown to induce neutralizing antibodies and protection against MERS-CoV [ 47 , 48 ]. Notably, the N-terminal domain (NTD) of the S protein binds to sialic acid and is important for MERS-CoV infection in certain cell types. Jiaming et al. showed that immunization with NTD-based vaccine also provides protection against MERS-CoV and induces potent humoral and cell-mediated immunity [ 49 ]. However, since SARS-CoV-2 NTD does not have the same sialic acid-binding function as MERS-CoV, NTD-based strategy might not be generalizable to SARS-CoV-2 vaccine development.
Apart from antigen design, several other factors also affect the efficacy of protein subunit vaccines [ 16 ]. The expression system of protein influences the quality and quantity of protein subunit vaccines. Du et al. demonstrated that SARS-CoV RBD protein expressed by mammalian 293T cells induces stronger neutralizing antibody responses than RBD expressed by insect cells and E. coli , which is probably due to the native conformation and post-translational modification maintained in mammalian cellular system [ 43 ]. In addition, adjuvants play an important role in enhancing the immunogenicity of protein subunit vaccines. Zhang et al. have examined multiple adjuvants (Freund’s, aluminum, Monophosphoryl lipid A, Montanide ISA51 and MF59) in conjugation with MERS-CoV RBD and found that MF59 best potentiate the protein to elicit neutralizing antibodies and protective effects [ 50 ]. Their data provide a good starting point for optimizing adjuvants for SARS-CoV-2 subunit vaccines. Moreover, the immunization route of the subunit vaccine can also affect its potency, and in combination with different antigen and adjuvants, the optimized vaccination pathway may change. For example, Li et al. demonstrated that for SARS-CoV S and S1 subunit vaccine, intramuscular (I.M.) route induces stronger antibody responses than subcutaneous (S.C.) route, while Lan et al. showed that S.C. route is preferable over I.M. injection for Freund’s and CpG-adjuvanted MERS-CoV RBD vaccine [ 23 , 51 ]. Therefore, the ideal route might need to be customized for SARS-CoV-2 subunit vaccines.
Virus-like particle vaccine
Virus-like particles (VLPs) are self-assembled viral structural proteins that mimic the conformation of native viruses but lack the viral genome. Compared with protein subunit vaccines, VLP vaccines present epitope in conformation that is more similar to the native virus, leading to better immunization responses. In addition, compared to whole virus vaccines, the production of VLP vaccines does not involve live virus or inactivation steps, which makes them safer vaccine candidates. The highly repetitive antigenic surface of VLP vaccines also help induce stronger antibody response by efficiently cross-linking B-cell surface receptors. Up to now, VLP vaccines have been commercialized for the protection against human papillomavirus (Cervarix™ and Gardasil®) and hepatitis B virus (Engerix® and Recombivax HB®) [ 52 ].
Few SARS-CoV and MERS-CoV VLP vaccines have been reported so far. For SARS-CoV, Lokugamage et al. have demonstrated that chimeric VLPs composed of SARS-CoV S protein and mouse hepatitis virus E, M and N proteins can induce neutralizing antibody responses and reduce SARS-CoV virus titer in mice lung after viral challenge [ 53 ]. In addition, another study performed by Liu et al. showed that chimeric VLPs consisting of SARS-CoV S protein and influenza virus M1 protein can induce neutralizing antibodies and provide protection against lethal challenge in mice [ 54 ]. However, one study used the same chimeric VLPs as Lokugamage et al. and showed that this VLP vaccine can lead to pulmonary immunopathology on challenge with SARS-CoV [ 37 , 53 ]. Therefore, potential adverse effects of coronavirus VLP vaccines should be monitored. For MERS-CoV VLP vaccines, Wang et al. have shown that VLPs containing MERS-CoV S, E and M proteins can induce specific antibody response and Th1-mediated cellular immunity in rhesus macaques [ 55 ]. The same research group developed another chimeric VLP vaccine containing the fusion of the receptor-binding domain (RBD) of MERS-CoV S protein and the canine parvovirus (CPV) VP2 structural protein [ 56 ]. They showed that this VLP vaccine induces MERS-CoV-specific antibody response and T-cell immunity in mice [ 56 ]. These studies suggested that VLP vaccines hold the potential for clinically effective coronavirus vaccines.
DNA vaccine
DNA vaccines contain genes encoding viral antigenic components that are expressed by plasmid vectors and delivered into cells through electroporation. Compared with other vaccine technologies, DNA vaccines offer a fast and flexible platform for vaccine development and production, making it an attractive technology to combat emerging epidemics like SARS-CoV-2. In addition, antigen production of DNA vaccines happens in the target cells, which helps recapitulate the native conformation and post-translational modification of viral antigens. However, an important drawback of DNA vaccines is their limited immunogenicity due to their inability to spread and amplify in vivo. Therefore, it is important to consider strategies that can enhance the potency of DNA vaccines, such as adding adjuvant or using a prime-boost regimen. Besides, the genomic integration of DNA vaccines into the host chromosome is another biosafety concern, which may lead to mutagenesis and oncogenesis [ 57 ]. Even though previous studies have shown that the risk of vaccine plasmid insertion into the host chromosome is pretty low, the FDA and the WHO still recommends integration studies be included as part of the safety program of DNA vaccines [ 58 , 59 ].
Several DNA vaccine candidates have been reported for SARS-CoV, including the S-, M-, and N protein-based vaccines [ 60 , 61 , 62 , 63 , 64 ]. Although all of them can generate a certain level of antibody and cell-immune responses, only S protein-based DNA vaccine has been shown to induce protective effect against SARS-CoV infection, probably due to the indispensable role of S protein in receptor binding [ 60 ]. Yang et al. has demonstrated that immunization with DNA encoding full-length S protein, S protein lacking part of cytoplasmic domain, S protein lacking both cytoplasmic and transmembrane domains can all induce neutralizing antibodies and T-cell immune responses, as well as providing protective effect in mice [ 60 ]. This promising result leads to a following phase I clinical trial based on SARS-CoV full-length S protein DNA vaccine, which showed that the vaccine was well-tolerated in patients and can induce neutralizing antibodies and T cell responses in healthy adults [ 65 ]. Furthermore, two studies have made use of prime-boost strategy to enhance the potency of S protein-based SARS-CoV DNA vaccine. Zakhartchouk et al. reported that the combination of DNA and whole-inactivated SARS-CoV vaccines can increase the magnitude of antibody response as well as inducing a more desirable Th1-skewed immune response [ 66 ]. Woo et al. demonstrated that using DNA vaccine priming plus E. coli expressed recombinant S protein booster can also induce higher neutralization titers than DNA or protein subunit vaccine alone [ 67 ].
Similar to SARS-CoV, several studies on MERS-CoV DNA vaccines have demonstrated optimistic results. Muthumani et al. reported that a full-length S protein-based MERS-CoV DNA vaccine can induce potent cellular immunity and antigen-specific neutralizing antibodies in mice, macaques, and camels, and macaques vaccinated with this DNA vaccine were protected against MERS-CoV challenge without demonstrating any clinical or radiographic signs of pneumonia [ 68 ]. Building on these encouraging data, a phase I clinical trial based on this MERS-CoV DNA vaccine (GLS-5300, or INO-4700) has been completed [ 69 ]. The results showed that GLS-5300 is well tolerated with no vaccine-associated serious adverse events, and immunization with GLS-5300 induces durable immune responses in 85% of participants after two vaccinations [ 69 ]. These data support further development of the GLS-5300 vaccine. Notably, a SARS-CoV-2 DNA vaccine candidate, INO-4800, is based on the same design as GLS-5300, and this vaccine is now in phase I/II clinical trial (NCT04447781 and NCT04336410) [ 70 ]. In addition, another MERS-CoV vaccine study using full-length S protein DNA priming plus S1 protein subunit booster elicits robust serum-neutralizing activity against several MERS-CoV strains in mice and rhesus macaques [ 47 ]. Immunizing rhesus macaques with this DNA prime/protein boost vaccine confers protection against MERS-CoV-induced radiographic pneumonia, supporting this strategy as a promising approach for MERS-CoV vaccine development [ 47 ]. Aside from full-length S, S1 subunit is also a good target for MERS-CoV DNA vaccine. One study performed by Al-Amri et al. has compared the immunogenicity of full-length S-based (pS) and S1-based (pS1) MERS-CoV vaccine using the same expression vector [ 71 ]. They found that pS1 immunization elicited a balanced Th1/Th2 response and generally higher levels of all IgG isotypes compared to pS vaccination, which may be explained by the fact that the transmembrane domain-lacking S1 subunit is secreted more efficiently to the extracellular space and therefore result in greater uptake by antigen-presenting cells [ 71 ]. This study suggested that S1 might be a better target than full-length S for MERS-CoV DNA vaccine [ 71 ].
Taken together, DNA vaccines encoding full-length S or S1 protein have demonstrated encouraging results to fight against SARS-CoV and MERS-CoV. The same strategy is likely to be generalizable to SARS-CoV-2 DNA vaccine considering the biological similarity.
Viral vector vaccine
Viral vector vaccines are recombinant viruses that encode antigens of interest in an unrelated modified virus. They deliver antigen into the cells mimicking natural infection, so they induce strong antigen-specific cellular and humoral immune responses per se, thereby obviating the need for additional adjuvants. In addition, viral vectors are able to accept large insertions in their genome, providing a flexible platform for antigen design. Despite these advantages, there are several drawbacks. The manufacturing process for viral vector vaccines is more complicated than other approaches, including the optimization of cellular systems and the exclusion of contaminants, which can greatly affect the efficiency of viral vectors [ 57 ]. Moreover, recombinant viruses carry the risk of integrating their genome into the human host, so additional biosafety assessment will be required before entering clinical trials. Finally, if the chosen viral vector can infect the general populations, the pre-existing immunity on the viral vector could dampen the induced immune response, which has been seen in adenovirus- and measle virus-based vaccines [ 72 , 73 ].
Similar to DNA and protein subunit vaccines, most viral vector coronavirus vaccines target the S antigen. Numerous viral vectors have been used to develop SARS-CoV and MERS-CoV vaccines, which have been described in detail in previous review articles [ 74 , 75 ]. In the following sections, we will highlight vaccines based on adenovirus, modified vaccinia virus Ankara and Venezuelan equine encephalitis virus, which are the most well studied viral vector platforms for coronavirus vaccines. We will also briefly introduce other recombinant viral platforms that are being actively developed for coronavirus vaccines.
SARS-CoV viral vector vaccine
Adenovirus is a popular viral vector vaccine that has been tested in clinical trials for a wide variety of diseases, and several studies have also examined the efficacy of adenovirus-based SARS-CoV vaccine. The feasibility of SARS adenovirus vector vaccine was first demonstrated in two study by Gao et al. and Liu et al. [ 76 , 77 ]. They showed that adenoviral vector expressing S1 fragment can induce neutralizing antibodies in monkeys and rats, respectively, but neither studies showed evidences of in vivo protection against SARS-CoV challenge [ 76 , 77 ]. Later on, See et al. compared the efficacy of SARS-CoV S protein expressing adenovirus vaccine with the whole inactivated SARS-CoV vaccine [ 78 ]. They found that both vaccines induce protective effects in mice against SARS-CoV challenge, though the neutralizing antibody response is weaker in adenovirus-based vaccine than whole-inactivated virus vaccine [ 78 ]. Besides, Kobinger et al. have also tested a prime-boost regimen of S protein-expressing human adenovirus type 5 and chimpanzee derived adenoviruses in ferrets [ 79 ]. Their results showed that this vaccine leads to a substantial reduction in viral load and prevents pneumonia in ferrets after SARS-CoV challenge [ 79 ]. All these results have encouraged subsequent development of adenovirus-based MERS and COVID-19 vaccines.
Modified vaccinia virus Ankara (MVA) is another well-established vaccine platform to combat emerging infectious diseases [ 80 ]. Bisht et al. has shown that intranasal or intramuscular immunization with highly attenuated MVA containing full-length S gene induces both neutralizing antibody responses and protective immunity in mice, evident by reduced virus titer in mice lung post SARS-CoV challenge [ 81 ]. Another study performed by Chen et al. demonstrated that recombinant MVA expressing SARS-CoV S protein elicits neutralizing antibodies in mice, ferrets, and monkeys, but they didn’t show any protection experiment data in this study [ 82 ]. However, another two studies performed by Weingartl et al. and Czub et al. showed that MVA vaccine expressing SARS-CoV S protein does not provide protective effect in ferrets, and it even induces inflammatory responses and focal necrosis in the liver [ 83 , 84 ]. Therefore, potential adverse effects need to be considered for MVA-based SARS-CoV S protein vaccine.
For Venezuelan equine encephalitis (VEE) virus-based SARS-CoV vaccine, Deming et al. has reported that VEE virus replicon particles (VRP) expressing S protein provides complete short- and long-term protection against homologous strain challenge in young and senescent mice [ 85 ]. To further improve the efficacy of VEE virus-based vaccine against heterologous SARS-CoV challenge, Sheahan et al. has improved the immunogenicity of VRP S protein vaccine by substituting an attenuated VEE glycoprotein with its wild-type counterpart, and their result showed that the improved VRP S protein vaccine can protect aged mice from heterologous SARS-CoV challenge [ 86 ].
Several additional viral vectors have also shown promising results for SARS-CoV vaccines. Two studies from Buchholz et al. and Bukreyev et al. have used an attenuated parainfluenza virus as vector to express SARS-CoV S protein, showing that parainfluenza-based vaccine can induce neutralizing antibody responses and protective effect against SARS-CoV challenge in hamsters and monkeys [ 30 , 87 ]. Besides, attenuated vesicular stomatitis virus (VSV) have also been tested as SARS-CoV vaccine vectors by Kapadia et al. [ 88 ]. Their results showed that immunization with recombinant VSV expressing S protein can induce SARS-neutralizing antibodies and is able to protect mice from SARS-CoV infection [ 88 ].
MERS-CoV viral vector vaccine
Several adenovirus-based MERS-CoV vaccines have been developed. Human adenovirus type 5 (Ad5) and type 41 (Ad41) expressing MERS-CoV S or S1 protein have been shown to induce neutralizing antibodies in mice [ 89 , 90 ]. However, the protection effect of Ad5- and Ad41-based MERS vaccines have not been evaluated [ 89 , 90 ]. Notably, Ad5-MERS-S vaccine has been used in combination with S protein nanoparticles [ 91 ]. Heterologous immunization by priming with Ad5/MERS and boosting with spike protein nanoparticles has demonstrated not only protective effect in hDPP4-transduced mice against MERS-CoV challenge, but also more balanced Th1/Th2 responses than Ad5- or nanoparticles-alone homologous prime-boost vaccines [ 91 ]. The Ad5 vector has already been applied to SARS-CoV-2 vaccine development, and promising results have been demonstrated in phase I and II clinical trials [ 92 , 93 ].
Besides, chimpanzee adenovirus has been employed as viral vector with an aim to overcome the pre-existing immunity problem of human adenoviruses. A MERS-CoV S protein vaccine based on a chimpanzee adenoviral vector (ChAdOx1) was shown to induce high levels of neutralizing antibodies and cell-mediated immune in mice, and to protect hDPP4-transduced mice from lethal MERS-CoV challenge [ 94 , 95 ]. In addition, ChAdOx1-MERS vaccine has also been demonstrated to reduce viral load in dromedary camels and provide protective immunity in rhesus macaques [ 96 , 97 ]. Given these promising preclinical results, the ChAdOx1-MERS vaccine has entered a phase I clinical trial, and the trial result showed that ChAdOx1-MERS was safe and well tolerated at all tested doses, and a single dose was capable of inducing both humoral and cellular responses against MERS-CoV [ 98 ]. Notably, the same research team has applied ChAdOx1 platform to SARS-CoV-2 vaccine development and their product AZD1222 (or ChAdOx1-nCoV-19) is now a leading player of the COVID-19 vaccine race [ 99 ].
The vaccine of modified vaccinia virus Ankara (MVA) expressing full-length MERS-CoV S protein has been reported to induce not only virus-neutralizing antibody responses and MERS-CoV-specific CD8+ T cell response, but also provide protective effect against MERS-CoV in DPP4-transduced mice [ 100 ]. Furthermore, dromedary camels immunized with this MVA-based MERS-CoV S protein vaccine generate neutralizing antibodies and show less virus excretion after MERS-CoV infection [ 101 ]. Since camel is a major animal reservoir for MERS-CoV, this vaccine provides an opportunity to effectively control camel-to-human transmission [ 101 ]. Finally, a phase I clinical trial showed that MVA-MERS-S vaccine has a favorable safety profile, and homologous prime–boost immunization of MVA-MERS-S vaccine induces humoral and cell-mediated immune responses against MERS-CoV, which supports testing MVA-MERS-S vaccine in a larger population [ 102 ].
MERS-CoV vaccines dependent on Venezuelan equine encephalitis (VEE) virus have also been studied. Agnihothram et al. have demonstrated that VEE virus replicon particles (VRP) expressing MERS-CoV S protein can induce neutralizing antibodies in young and aged mice [ 103 ]. Another study from Zhao et al. found that VRP-based MERS N protein vaccine can induce memory CD4+ immune response and provide protective immunity against MERS-CoV in hDPP4-transduced mice [ 28 ]. Since N protein is more conserved than S protein across different coronavirus species, their approach might hold the potential to develop a universal T cell-inducing coronavirus vaccine [ 28 ].
Several other vaccine platforms have been applied for the development of MERS-CoV vaccine. Measle virus- and rabies virus-based MERS-CoV S protein vaccines have been shown to induce neutralizing antibodies and provide protective effect against MERS-CoV in hDDP4-transduced mice [ 104 , 105 ]. Newcastle disease virus and vesicular stomatitis virus vectors have also been employed as S protein-expressing MERS vaccines [ 106 , 107 ]. However, only in vitro neutralization data have been provided and no in vivo protection data has been demonstrated for these two vaccines [ 106 , 107 ].
In summary, SARS-CoV and MERS-CoV vaccines based on many viral vectors, including adenovirus, modified vaccinia virus Ankara, Venezuelan equine encephalitis virus, parainfluenza virus, vesicular stomatitis virus, Measle virus, and rabies virus, have been shown to elicit protective immunity against viral challenges. Some of these viral vectors has already become promising candidate platforms for the development of SARS-CoV-2 vaccine.
Whole inactivated vaccine
Whole inactivated vaccines are composed of chemically or radiationally inactivated virions. They contain a full repertoire of immunogenic components of the original virus, and compared with attenuated viruses, they carry no risk of viral reactivation if properly inactivated. Although safer than live attenuated vaccines, the immunogenic epitopes of inactivated viruses may be structurally deformed during the inactivation process, which can undermine the protection they may provide. Moreover, both SARS-CoV and MERS-CoV whole inactivated vaccines have been reported to induce eosinophil-related lung pathology [ 36 , 37 ]. These disadvantages make whole inactivated vaccines a less attractive strategy for coronavirus vaccine development.
During the early development of SARS-CoV vaccines, inactivated whole virus was once a leading strategy. Studies have shown that UV- and formaldehyde-inactivated SARS-CoV can induce neutralizing antibody response, and a phase I clinical trial using β-propiolactone-inactivated SARS-CoV vaccine demonstrated that it is safe, well-tolerated, and can elicit SARS-CoV-specific neutralizing antibodies [ 108 , 109 , 110 ]. However, later studies found that a UV-formaldehyde doubly inactivated SARS-CoV vaccine, either unadjuvanted or alum-adjuvanted, provides incomplete protection in mice and induces eosinophilic pulmonary inflammatory response upon SARS-CoV challenge [ 36 ]. Similarly, gamma-irradiated MERS-CoV vaccine adjuvanted with alum or MF59 also induces eosinophil-related lung pathology after virus challenge, despite its ability to induce neutralizing antibodies [ 111 ]. These results have dampened the enthusiasm of whole-inactivated coronavirus vaccines. Nevertheless, recently two studies have revealed that UV-inactivated SARS-CoV adjuvanted with Toll-like receptor agonists, and formaldehyde-inactivated MERS-CoV adjuvanted with alum and unmethylated CpG, can reduce or even prevent Th2-skewed lung pathology after challenge [ 38 , 112 ]. These results demonstrated that with an appropriate combination of inactivation method and adjuvants, the whole inactivated virus is still a viable option for coronavirus vaccine development.
Live attenuated vaccine
Live attenuated vaccines are live viruses weakened by deleting or mutating the pathogenic component of the viral genome. Similar to whole inactivated vaccines, they possess nearly the full immunogenic components of the original virus. Furthermore, they preserve the native conformation of viral antigens and present antigens to the immune system as in natural infections. Therefore, live attenuated vaccines are the most immunogenic kind of vaccine and have a long history of success in controlling a variety of infectious diseases [ 113 ]. However, live attenuated vaccines also carry a higher risk than other types of vaccines, including the possibility of reversion to a virulent state and the danger of persistent infection in immunocompromised patients. Therefore, biosafety of live attenuated vaccines needs to be carefully evaluated before proceeding to clinical use.
Although a few SARS-CoV and MERS-CoV live attenuated vaccines have demonstrated efficacy in animal models, none of them have proceeded to clinical trials [ 114 , 115 , 116 , 117 ]. The envelope (E) protein, besides its structural roles, has a major role in inflammasome activation and is associated with exacerbated inflammation in the lung [ 118 ]. As a result, the deletion of E protein can lead to the decreased virulence of coronavirus [ 119 ]. Lamirande et al. has reported that SARS-CoV mutants lacking the E gene can induce protective effects in hamsters against SARS-CoV challenge [ 114 ]. In addition, nonstructural protein 16 (nsp16) is another viable target for the coronavirus vaccine. Nsp16 encodes ribose 2′- O -methyltransferase that is required for 5′ capping of viral RNA [ 120 ]. This methylation helps coronavirus avoid the activation of type I interferon-dependent innate immune response by viral RNA, and therefore nsp16 deletion attenuates virulence [ 120 ]. Both SARS-CoV and MERS-CoV nsp16 mutant vaccines have been reported to provide protection against challenge [ 115 , 116 ]. Moreover, nonstructural protein 14 (nsp14), which encodes exoribonuclease (ExoN) involved in RNA proofreading during replication, is also an useful target for live attenuated coronavirus vaccine [ 121 ]. The loss of ExoN will cause a profound decrease in replication fidelity, and lead to attenuation of coronavirus pathogenesis [ 121 ]. Graham et al. has shown that ExoN deletion can reduce SARS-CoV virulence in young, aged, immunocompromised mice, and ExoN-deleted SARS-CoV vaccine can induce a protective effect against challenge in these mice [ 117 ]. In sum, all the targets mentioned above serve as potential strategies for the development of live attenuated SARS-CoV-2 vaccine.
Recent progress on SARS-CoV-2 vaccine development
Compared with SARS and MERS, which tended to resolve spontaneously after regional outbreak, the worldwide magnitude of the COVID-19 pandemic has made development of vaccine an unprecedented urgency. This urgent need has led to many different approaches in vaccine development considerations. First of all, unconventional vaccine platforms, such as nucleic acid vaccines and viral vector vaccines, are becoming the leading players in the race of COVID-19 vaccine development due to their ability to be developed using sequence information alone [ 122 ]. These new platforms are therefore highly adaptable to emerging pathogens, and their safety profiles have already been well examined in recent influenza, Ebola and Zika outbreaks [ 57 ]. Secondly, the clinical development process of COVID-19 vaccine has been accelerated by executing trials in parallel rather than following a linear sequence of steps. For example, multiple COVID-19 vaccine candidates directly entered clinical trials before having preclinical data in animal models, and many vaccine trials have adopted an integrated phase I/II or phase II/III approach to save time [ 123 ]. Last but not least, in order to meet the massive global need of COVID-19 vaccine, vaccine developing companies, especially the front runners, are ramping up their manufacturing capacity to the scale of ~ 1 billion doses per year [ 124 , 125 , 126 ]. Governments from the United States and several other countries are also playing an important role in funding the scale-up of potentially effective vaccines [ 127 , 128 , 129 ].
In this section, we will discuss the latest preclinical and clinical development of COVID-19 vaccines (as of Oct 26, 2020). We will highlight representative COVID-19 vaccines from each major vaccine platform that have published clinical data (Table 2 ).
Up to now, there have been 13 SARS-CoV-2 protein subunit vaccines entering clinical trials [ 130 ]. Among these vaccines, a leading company Novavax, with its NVX-CoV2373 vaccine, has entered a phase IIb trial in South Africa (NCT04533399) and a phase III trial in the UK (2020-004123-16). NVX-CoV2373 contains a prefusion stabilized full-length spike protein adjuvanted with their proprietary saponin-based adjuvant [ 131 , 132 ]. In a preclinical trial, the vaccine induced neutralizing antibodies and prevented viral replication in the respiratory tract in macaques challenged with the virus [ 131 ]. The vaccine also induced binding and neutralizing antibodies in all participants in the phase I trial [ 132 ]. In their phase I trial, they also observed a dose sparing effect by the adjuvant. They found that both adjuvanted 5 ug and 25 ug dose regimens induced significantly high titers of neutralizing antibody compared to the placebo group and the 25 ug dose without adjuvant group [ 132 ]. Another vaccine that has entered the phase II trial is Anhui Zhifei Longcom’s recombinant new coronavirus vaccine (NCT04466085). Instead of using the full-length S protein, Anhui Zhifei Longcom’s vaccine only contains the RBD of the SARS-CoV-2 S protein. However, no further design or data has been provided so far. For the other candidate SARS-CoV-2 protein subunit vaccines, most of them also utilize either full-length S protein or the RBD of S protein as their vaccine antigen. Notably, one recent study has described a generalizable strategy to enhance the immunogenicity of protein subunit coronavirus vaccines [ 133 ]. They identified a disulfide-linked dimeric form of MERS-RBD that is significantly more immunogenic and protective than its conventional monomeric counterpart [ 133 ]. They applied the same strategy to SARS-CoV-2 and has demonstrated a 10–100-fold enhancement of neutralizing antibody titers [ 133 ]. Therefore, this framework of immunogen design could be universally applicable to all protein subunit coronavirus vaccines in the future.
There are 4 DNA vaccines for SARS-CoV-2 currently under clinical trials [ 130 ]. Among these developers, Inovio is a leading company that has published results on MERS-CoV and SARS-CoV-2 DNA vaccines. Inovio’s SARS-CoV-2 DNA vaccine INO-4800 encodes the full length S protein and is administered intradermally with a hand-held device CELLECTRA to electroporate the skin cell [ 70 , 134 ]. Having experience in the phase I/IIa trial of their MERS vaccine (INO-4700), they are using the same platform for the SARS-CoV-2 vaccine INO-4800 [ 69 , 70 ]. They have demonstrated that the vaccine induces neutralizing antibodies and Th1-skewed immune responses in animal models including mice, guinea pigs, and rhesus macaques [ 70 , 135 ]. The vaccine is now in two phase I/II trial (NCT04447781 and NCT04336410). The interim analysis of the two phase I trials showed it induced humoral and T cell immune responses in 94% participants after two doses while only caused adverse events of grade 1 or below [ 136 ].
RNA vaccine
Although there were no RNA vaccine studies for SARS-CoV or MERS-CoV in the past two decades, there have already been 6 novel RNA vaccines reaching clinical trials for SARS-CoV-2 since the outbreak of COVID-19 [ 130 ]. RNA vaccines consist of viral antigen-encoding messenger RNAs that can be translated by human cells to produce antigenic proteins and stimulate the immune system. RNA vaccines are usually delivered in complex with additional agents, such as protamine or lipid- and polymer-based nanoparticles, to increase its efficacy [ 137 ]. Similar to DNA vaccines, RNA vaccines have the advantages of being highly adaptable to new pathogens and being able to recapitulate the native conformation and modifications of antigenic proteins. Furthermore, compared with DNA vaccines, RNA vaccines have some additional benefits. Unlike DNA, RNA does not interact with host-cell DNA and therefore obviate the risks of genomic integration. Besides, RNA vaccines can be given through multiple routes including traditional intravenous injection, whereas DNA vaccines need to be administered via special devices like electroporation or gene gun. Nevertheless, RNA vaccines do have some drawbacks. Exogenous RNA can activate interferon-mediated antiviral immune response and lead to stalled translation and mRNA degradation, which suppress the efficacy of RNA vaccines [ 138 ]. In addition, interferon signaling is associated with inflammation and potential autoimmunity [ 139 ]. Even though there have not been severe cases of RNA vaccine-induced autoimmune diseases, it is important to carefully evaluate this potential adverse effect.
Moderna and BioNTech/Pfizer are the two leading developers for a SARS-CoV-2 RNA vaccine. Moderna's mRNA-1273 vaccine encodes a stabilized prefusion spike trimer, in which they substituted the amino acids at 986 and 987 with proline to stabilize the spike protein in its prefusion conformation [ 140 ]. The nucleotides of the mRNA were also modified not only to increase its translation and half-life but also to prevent activation of interferon-associated genes upon entering the cell [ 140 ]. The preliminary report for their phase I clinical trial showed that: (1) neutralizing antibodies were detected in all 45 patients after two doses of immunization; (2) antibody titers of immunized patients were higher than convalescent serum after two doses of vaccination; (3) Th1-biased immune responses were observed in immunized patients [ 140 ]. There were some cases of systemic adverse events after the second dose of vaccination, but no grade 4 adverse events were observed [ 140 ]. They concluded that 100 ug can induce a satisfactory immune response and thus will continue to use 100 ug dosage in phase III clinical trial (NCT04470427) [ 140 ]. In addition, they also expanded the same phase I trial to include 40 elderly participants with their age older than 55 years old [ 141 ]. Their result demonstrated that 100 ug dose of mRNA-1273 induced higher binding- and neutralizing-antibody titers than the 25 ug dose, and the adverse events associated with mRNA-1273 were mild or moderate in these elderly participants [ 141 ]. On Nov 16, 2020, Moderna revealed the first interim analysis of their phase III trial (NCT04470427) [ 142 ]. Their result showed that among 95 people who developed symptomatic COVID-19 after volunteering in this trial, only 5 of them were from the mRNA-1273 group, and the rest 90 cases were from the placebo group, resulting in a estimated vaccine efficacy of 94.5% [ 142 ]. In addition, there were 11 volunteers who developed severe COVID-19 symptoms, and their analysis showed that all 11 cases were in the placebo group and none in the mRNA-1273 group [ 142 ]. Their concurrent safety review also did not notice any significant safety concern [ 142 ]. Therefore, their promising result suggested that the mRNA-1273 vaccine is safe and effective in preventing symptomatic COVID-19.
BioNTech and Pfizer’s mRNA vaccine has four candidates, BNT162b1, BNT162b2, BNT162a1 and BNT162c2. BNT162b1 and BNT162b2 are both nucleoside modified mRNA (modRNA) vaccine [ 143 ]. BNT162b1 encodes a trimerized RBD of spike protein while BNT162b2 encodes a full-length spike protein [ 143 ]. On the other hand, BNT162a1 is a uridine mRNA (uRNA)-based vaccine and BNT162c2 is a self-amplifying mRNA (saRNA)-based vaccine [ 143 ]. Up to now, BioNTech and Pfizer have published two BNT162b1 phase I/II trial results that were conducted in Germany (NCT04380701) and the US (NCT04368728), respectively [ 144 , 145 ]. Both studies showed that the two-dose regimen of BNT162b1 elicited RBD-binding and neutralizing antibodies with titers above convalescent human serum [ 144 , 145 ]. Analysis of cell-mediated immune responses showed Th1-skewed response in most participants, as demonstrated by the detection of IFNγ, IL-2 and IL-12 but not IL-4 in their assay [ 144 , 145 ]. Although the German trial and the US trial used different dosages of vaccine, the two trials agreed with each other and showed that a regimen of 30–50 ug on day 1 and day 22 is able to elicit favorable immune response without severe adverse effects [ 144 , 145 ]. Following these two papers, they also published another study comparing the vaccination responses between BNT162b1 and BNT162b2 [ 146 ]. BNT162b1 and BNT162b2 were shown to induce similar neutralizing titers in younger and older adults [ 146 ]. However, BNT162b2 had less systemic reactogenicity in older adults [ 146 ]. Therefore, they decided to move forward with BNT162b2 instead of BNT162b1 into a phase III clinical trial (NCT04368728). On Nov 18, 2020, Pfizer and BioNTech announced the efficacy analysis of their phase III clinical trial (NCT04368728) after meeting all primary efficacy endpoints [ 147 ]. Their evaluation showed that BNT162b2 is 95% effective against COVID-19 [ 147 ]. This result was based on analyzing 170 confirmed COVID-19 cases, of which 162 cases of COVID-19 were observed in the placebo group while 8 cases in the BNT162b2 group [ 147 ]. In addition, among 10 severe COVID-19 cases observed in this trial, 9 of them were in the placebo group and only 1 of them was in the BNT162b2 group [ 147 ]. Notably, the observed efficacy in the elderly people was over 94%, which would help protect the most vulnerable population against COVID-19 [ 147 ]. No serious safety concern was observed among 43,000 enrolled participants [ 147 ]. These data indicated BNT162b2 is another well-tolerated and efficacious COVID-19 vaccine.
Currently, there are 12 viral vector vaccines in clinical trials, and an additional 36 viral vector vaccines under preclinical development [ 130 ]. Many viral vector platforms that have been tested in SARS-CoV and MERS-CoV are being explored in COVID-19 vaccines, including adenovirus (both human and non-human primates), measles virus, modified vaccinia virus Ankara (MVA), parainfluenza virus, rabies virus and vesicular stomatitis virus (VSV) [ 130 ]. Surprisingly, Venezuelan equine encephalitis (VEE) virus, which has been extensively studied in SARS and MERS vaccine, hasn’t been tested in any COVID-19 vaccine studies yet. On the other hand, influenza virus vector, which hasn’t been explored for SARS and MERS viral vector vaccines, are now gaining popularity for the development of COVID-19 viral vector vaccine [ 130 ]. For COVID-19 viral vector vaccines that have entered clinical trials, 8 out of 12 are based on adenoviruses, and the four leading candidates in this platform are AZD1222 (or ChAdOx1 nCoV-19, developed by Astrazeneca and Oxford University), Gam-COVID-Vac (or Sputnik V, or rAd26S+rAd5-S, developed by Gamaleya Research Institute), Ad5 (developed by CanSino Biological Inc. and Beijing Institute of Biotechnology), and Ad26 (developed by Johnson & Johnson and Beth Israel Deaconess Medical Center) [ 130 ].
AZD1222 is a chimpanzee adenovirus-based viral vector vaccine (ChAdOx1) expressing SARS-CoV-2 spike protein [ 99 ]. This ChAdOx1 platform has been used to develop MERS-CoV vaccine, which has demonstrated promising preclinical and phase I clinical trial data [ 94 , 95 , 96 , 97 , 98 ]. The AZD1222 vaccine team published their phase I/II trial interim report in July 2020 and showed that AZD1222 can elicit S protein-specific antibody and T-cell response and induce neutralizing antibody in all participants after the prime-boost regimen [ 99 ]. No severe adverse effect has been observed [ 99 ]. Based on this promising data, AZD1222 launched phase II/III trials in UK (2020-001228-32) and phase III trials in Brazil (ISRCTN89951424), United States (NCT04516746), Russia (NCT04540393) and India (CTRI/2020/08/027170). In Sep 2020, the AZD1222 phase II/III trial in the UK was once put on hold for safety review because a participant has developed unexplained illness, but following later independent review in the UK determined that the trial is still safe and therefore the AZD1222 clinical trial resumed [ 148 , 149 ]. On Nov 23, 2020, Astrazeneca annouced the interim analysis of their clinical trial in UK (2020-001228-32) and Brazil (ISRCTN89951424) [ 150 ]. Their pooled result showed that AZD1222 has an average efficacy of 70%, based on analyzing a total of 131 COVID-19 cases from 11,636 volunteers [ 150 ]. Interestingly, one dose regimen showed 90% efficacy when AZD1222 was given as half first dose followed by a full second dose ( n = 2,741) [ 150 ]. On the other hand, two full dose regimen had only 62% efficacy ( n = 8,895) [ 150 ]. Due to the response discrepancy between different subgroups, additional trials may be needed to better determine the efficacy and the most suitable regimen of AZD1222. In addition, the Gam-COVID-Vac vaccine team has published their phase I/II trial results [ 151 ]. They conducted two different trials, with one using frozen formulation (NCT04436471) and the other using lyophilized formulation (NCT04437875) of the vaccine [ 151 ]. In both phase II trials, they tested their patients with heterologous prime-boost immunization of recombinant adenovirus type 26 vector encoding SARS-CoV-2 spike glycoprotein (rAd26-S) plus recombinant adenovirus type 5 vector encoding SARS-CoV-2 spike glycoprotein (rAd5-S) [ 151 ]. Their results showed that both frozen and lyophilized formulation of the vaccine induced potent neutralizing antibodies and CD4+ and CD8+ T-cell immune responses, with the immune response of frozen formulation being slightly stronger than the lyophilized formulation [ 151 ]. Both vaccines were safe and well-tolerated in all participants [ 151 ]. Now this vaccine is also entering phase III trial in Russia (NCT04530396) and Belarus (NCT04564716). On Nov 24, 2020, Gamaleya Research Institute announced the second interim analysis of Gam-COVID-Vac (or Sputnik V) phase III clinical trial (NCT04530396) [ 152 ]. Their result showed that Gam-COVID-Vac had a efficacy of 91.4% on Day 28 after the first dose, which was based on analyzing 39 confirmed cases among 18,794 volunteers [ 152 ]. They also revealed that on Day 42 after the first dose (Day 21 after the second dose), the vaccine efficacy was even above 95% [ 152 ]. There were no unexpected adverse effect documented during the trial [ 152 ]. These promising results suggested that Gam-COVID-Vac is safe and effective in preventing COVID-19. Furthermore, the Ad5 vaccine team, whose vaccine is based on human adenovirus 5, has also published their clinical data [ 92 , 93 ]. In their phase II study, Ad5-vectored COVID-19 vaccine induces significant neutralizing antibodies and T-cell mediated immune response after single immunization [ 93 ]. They tested two dosage, 1 × 10 11 and 5 × 10 10 viral particles, and showed that the 5 × 10 10 dose causes less severe adverse reactions without compromising the immunogenicity [ 93 ]. Now this vaccine has advance to two phase III global multi-centered clinical trials (NCT04526990 and NCT04540419). Finally, Johnson & Johnson’s Ad26-based COVID-19 vaccine has also entered phase III clinical trial (NCT04505722), but no data from its earlier trial has been reported yet.
Currently, there are 7 whole inactivated COVID-19 vaccine in clinical trials [ 130 ]. From the previous experience of SARS-CoV and MERS-CoV vaccine development, whole inactivated virus can induce adverse effect such as eosinophil-related lung immunopathology in preclinical models [ 36 , 37 ]. Even though no serious adverse effect has been reported for whole-inactivated COVID-19 vaccine, it is important for the research community to keep this in mind and carefully evaluate potential adverse effects. For all ongoing trials of whole inactivated COVID-19 vaccine, three of them have publicly reported their preclinical or clinical data. SinoVac Inc. developed CoronaVac (also known as PiCoVacc), which is a beta-propiolactone inactivated, Vero cell line propagated whole virus vaccine originated from a patient-derived CN-2 SARS-CoV-2 virus strain [ 153 ]. In their preclinical study, PiCoVacc induces broad neutralizing antibodies against 10 representative SARS-CoV-2 strains in mice, rats, and non-human primates [ 153 ]. Immunizing macaques with three doses of PiCoVacc provides them with protective immunity against SARS-CoV-2 challenge without causing any antibody-dependent enhancement effect [ 153 ]. Following their preclinical study, CoronaVac has completed two phase I/II trials (NCT04383574 and NCT04352608, result not yet published) and is now starting phase III clinical trial in Brazil (NCT04456595), Indonesia (669/UN6.KEP/EC/2020) and Turkey (NCT04582344). In addition, Sinopharm Inc. and Wuhan Institute of Biological Products have developed a different COVID-19 inactivated virus vaccine (no specific product name). In this vaccine, WIV04 strain was isolated from a COVID-19 patient in Wuhan, propagated in Vero cells, and followed by two rounds of beta-propiolactone inactivation [ 154 ]. They tested three different dosage and three different injection timelines in their phase I and phase II studies, and their phase I/II interim report showed that patients receiving different vaccination regimen all had demonstrated neutralizing antibodies and with only a low rate of adverse reactions [ 154 ]. Now they have launched a phase III clinical trial in United Arab Emirates (ChiCTR2000034780) and Kuwait (ChiCTR2000039000). Finally, Sinopharm Inc. also collaborated with Beijing Institute of Biological Products to develop another COVID-19 inactivated virus vaccine BBIBP-CorV [ 155 , 156 ]. The manufacturing process of BBIBP-CorV is very similar to the other vaccine Sinopharm Inc. produced, except that BBIBP-CorV used a different HB02 strain rather than WIV04 strain [ 155 ]. They have tested BBIBP-CorV in preclinical models and showed that two-dose immunization of BBIBP-CorV can protect rhesus macaques from SARS-CoV-2 challenge [ 155 ]. Following this, they completed a clinical phase I/II trial with BBIBP-CorV and demonstrated that BBIBP-CorV is safe and well-tolerated in all tested doses in two age groups [ 156 ]. Furthermore, their immunogenicity result showed that humoral responses against SARS-CoV-2 were induced in all vaccine recipients 42 days after immunization [ 156 ]. Now this vaccine is under phase III clinical trial in United Arab Emirates (ChiCTR2000034780) and Argentina (NCT04560881).
Other vaccine platforms
There are also several other COVID-19 vaccine candidates using different technologies other than the platforms mentioned above. Virus-like particle-based vaccine, which has been demonstrated to induce humoral and cell-mediated immunity in SARS-CoV and MERS-CoV preclinical models, has one candidate COVID-19 vaccine in phase I clinical trial (NCT04450004) and 14 vaccine candidates under preclinical development [ 130 ]. However, none of the group has publicly reported their vaccine studies yet. Live attenuated vaccine, which has been shown to provide protective effect in SARS-CoV and MERS-CoV challenged mice, has 3 preclinical ongoing studies [ 130 ]. The higher risk of adverse effect has made live attenuated vaccine a less appealing choice for the time-sensitive race of COVID-19 vaccine development. Nevertheless, if successfully developed, live attenuated vaccine can provide the most potent protective effect due to its high similarity to natural infection.
In addition to these traditional platforms, scientists have also developed COVID-19 vaccines using unconventional approaches. Aivita Biomedical, Inc. has developed AV-COVID-19, which is an autologous dendritic cells vaccine loaded with SARS-CoV-2 antigens [ 157 ]. AV-COVID-19 is derived from patients’ own peripheral blood monocytes, then differentiated in vitro into dendritic cells, and incubated with SARS-CoV-2 antigens before injecting back into patients’ blood [ 157 ]. Now the company has launched a phase I/II clinical trial to evaluate its safety and efficacy profile in adults (NCT04386252). Besides, Symvivo Corporation has developed bacTRL-Spike, a live Bifidobacterium vaccine engineered to deliver synthetic plasmid DNA encoding spike protein from SARS-CoV-2. They have also registered a phase I clinical trial to examine the safety of this vaccine (NCT04334980). Moreover, a group from Nanjing University has found that a plant microRNA, MIR2911, can target SARS-CoV-2 by binding to their mRNA and blocking protein translation [ 158 ]. Their data showed that MIR2911 inhibited SARS-CoV-2 replication and accelerated negative conversion of infected patients [ 158 ]. Following their study, they are now launching a phase I clinical trial (ChiCTR2000031432) in China to evaluate the safety and tolerance of MIR2911 in patients.
Finally, people have been testing existing licensed vaccines and trying to repurpose them to combat COVID-19. It has been shown that tuberculosis vaccine bacillus Calmette–Guérin (BCG) can train innate immunity and induce nonspecific host defensive reaction against viral pathogens, including respiratory syncytial virus (RSV), influenza A virus and herpes simplex virus type 2 (HSV2) [ 159 , 160 , 161 , 162 ]. Additionally, an interesting study compared the national difference in COVID-19 impact and correlated it with national BCG vaccination policy [ 163 ]. They found that countries without universal policies of BCG vaccination have been more severely affected compared to countries with universal and long-standing BCG policies [ 163 ]. Based on these rationales, there have been at least 13 phase III clinical trials testing whether BCG vaccine can reduce the morbidity and mortality of healthcare workers (NCT04328441, NCT04327206, NCT04350931, NCT04348370, NCT04362124, NCT04369794, NCT04373291, NCT04379336, NCT04384549, NCT04439045, NCT04387409, NCT04417335, NCT04414267).
Additional considerations for SARS-CoV-2 vaccine development
Given the rapid transmission and asymptomatic spread of COVID-19, it is clear that an effective vaccine with global immunization coverage is required to bring people’s lives back to normalcy. However, even when an effective SARS-CoV-2 vaccine becomes available, the duration of vaccine-induced immunity is still largely unknown. Previous SARS studies have shown that SARS-specific IgG and neutralizing antibodies were only maintained for approximately 2 years in patients who recovered from SARS-CoV infection [ 164 , 165 ]. As a result, permanent immunity is less likely to be the case for COVID-19 vaccines, and a regular vaccination policy might be required in the future. In addition, it is still unclear what is the minimal neutralizing antibody titer that can provide protective effect against SARS-CoV-2 infection. It is believed that the higher neutralizing antibody vaccination induces, the better protective effect it will be. This is consistent with the observation that most COVID-19 reinfection cases only experience mild or no symptom during their first infection, which might not be sufficient to induce strong neutralizing antibodies [ 166 , 167 ]. Therefore, it is of great importance that further studies characterize the correlation between neutralizing antibody and protective effect to guide COVID-19 vaccine development. Last but not least, various mutations have been detected in the SARS-CoV-2 genome, with D614G mutation being the most prevalent one [ 168 ]. D614G is a missense point mutation in S protein that increases the infectivity of SARS-CoV-2 by decreasing S1 shedding and increasing S protein incorporation into virion [ 169 , 170 ]. Fortunately, D614G mutation does not prevent neutralizing antibodies from binding to SARS-CoV-2 and thus does not provide resistance to vaccination [ 170 ]. However, it is possible that such immune-escaping mutations appears in the future and makes COVID-19 vaccine development even more difficult.
Concluding remarks
Since the discovery of human coronaviruses in 1960s, new types of coronaviruses have kept emerging and have gradually become a serious threat to global public health. Even though there have been almost two decades since the first coronavirus outbreak, the scientific and medical community are not well prepared with effective weapons to combat these pathogens. One lesson we learned from this is that the financial and regulatory mechanism of current pharmaceutical market does not provide enough incentive to encourage vaccine development before a deadly outbreak happens. To make up for this, now academic institutions and companies all over the world are developing an explosive numbers of vaccine candidates with highly compressed clinical trial schedules. Fortunately, the biological and clinical lessons we learned from SARS-CoV and MERS-CoV researches, together with the vaccine development experience we gained from other diseases, have already guided us to come up with multiple promising candidate solutions. Besides, multiple therapeutic candidates targeting molecules in SARS-CoV-2 life cycle and human immune response against COVID-19 have also been rapidly explored, with Remdesivir and Dexamethasone being the two leading drugs that showed promising clinical evidences in shortening the time to recovery and decreasing mortality rates [ 171 , 172 ]. These treatment options can be complementary to SARS-CoV-2 vaccines to achieve overall mitigation of the COVID-19 pandemic. In conclusion, we hope countries all over the world, regardless of political ideologies, can unite and work together to achieve fast and successful COVID-19 vaccine development in the near future.
Availability of data and materials
Not applicable.
Abbreviations
Coronavirus Disease 2019
Coronavirus
Severe Acute Respiratory Syndrome Coronavirus
Middle East Respiratory Syndrome Coronavirus
Severe Acute Respiratory Syndrome Coronavirus 2
Polyproteins
Non-structural proteins
Necleocapsid
Receptor binding domain
Angiotensin-converting enzyme 2
Dipeptidyl peptidase-4
Endoplasmic reticulum
Antibody-dependent enhancement
N-terminal domain
Intramuscular
Subcutaneous
Virus-like particle
Canine parvovirus
Venezuelan equine encephalitis
Virus replicon particle
Human adenovirus type #
Chimpanzee Adenoviral Vector
Exoribonuclease
Modified RNA
Uridine mRNA
Self-amplifying RNA
Respiratory synctial virus
Herpes simplex virus
Centers-for-Disease-Control-and-Prevention. Human Coronavirus Types. 2020. https://www.cdc.gov/coronavirus/types.html .
van der Hoek L. Human coronaviruses: what do they cause? Antivir Ther. 2007;12(4 Pt B):651–8.
PubMed Google Scholar
World-Health-Organization. Summary of probable SARS cases with onset of illness from 1 November 2002 to 31 July 2003. Geneva: World-Health-Organization; 2003.
Google Scholar
World-Health-Organization. MERS situation update, January 2020. Geneva: World-Health-Organization; 2020a.
Del Rio C, Collins LF, Malani P. Long-term health consequences of COVID-19. JAMA. 2020. https://doi.org/10.1001/jama.2020.19719 .
Article PubMed PubMed Central Google Scholar
Li Q, Guan X, Wu P, Wang X, Zhou L, Tong Y, Ren R, Leung KSM, Lau EHY, Wong JY, Xing X, Xiang N, Wu Y, Li C, Chen Q, Li D, Liu T, Zhao J, Liu M, Tu W, Chen C, Jin L, Yang R, Wang Q, Zhou S, Wang R, Liu H, Luo Y, Liu Y, Shao G, Li H, Tao Z, Yang Y, Deng Z, Liu B, Ma Z, Zhang Y, Shi G, Lam TTY, Wu JT, Gao GF, Cowling BJ, Yang B, Leung GM, Feng Z. Early transmission dynamics in Wuhan, China, of Novel Coronavirus-Infected Pneumonia. N Engl J Med. 2020;382(13):1199–207.
Article CAS PubMed PubMed Central Google Scholar
Gandhi M, Yokoe DS, Havlir DV. Asymptomatic transmission, the Achilles’ Heel of current strategies to control covid-19. N Engl J Med. 2020;382(22):2158–60.
Article CAS PubMed Google Scholar
World-Health-Organization. Coronavirus disease (COVID-19) pandemic. Geneva: World-Health-Organization; 2020b.
Masters PS. The molecular biology of coronaviruses. Adv Virus Res. 2006;66:193–292.
Stadler K, Masignani V, Eickmann M, Becker S, Abrignani S, Klenk HD, Rappuoli R. SARS–beginning to understand a new virus. Nat Rev Microbiol. 2003;1(3):209–18.
Enjuanes L, Zuniga S, Castano-Rodriguez C, Gutierrez-Alvarez J, Canton J, Sola I. Molecular Basis of Coronavirus Virulence and Vaccine Development. Adv Virus Res. 2016;96:245–86.
NCBI-Reference-Sequence. SARS coronavirus Tor2, complete genome. 2020.
NCBI-Reference-Sequence. Middle East respiratory syndrome-related coronavirus isolate HCoV-EMC/2012, complete genome. 2020.
NCBI-Reference-Sequence. Severe acute respiratory syndrome coronavirus 2 isolate Wuhan-Hu-1, complete genome. 2020.
Du L, He Y, Zhou Y, Liu S, Zheng BJ, Jiang S. The spike protein of SARS-CoV–a target for vaccine and therapeutic development. Nat Rev Microbiol. 2009;7(3):226–36.
Wang N, Shang J, Jiang S, Du L. Subunit vaccines against emerging pathogenic human coronaviruses. Front Microbiol. 2020;11:298.
Snijder EJ, Decroly E, Ziebuhr J. The nonstructural proteins directing coronavirus RNA synthesis and processing. Adv Virus Res. 2016;96:59–126.
Cao Z, Liu L, Du L, Zhang C, Jiang S, Li T, He Y. Potent and persistent antibody responses against the receptor-binding domain of SARS-CoV spike protein in recovered patients. Virol J. 2010;7:299.
Article PubMed PubMed Central CAS Google Scholar
Zhong X, Yang H, Guo ZF, Sin WY, Chen W, Xu J, Fu L, Wu J, Mak CK, Cheng CS, Yang Y, Cao S, Wong TY, Lai ST, Xie Y, Guo Z. B-cell responses in patients who have recovered from severe acute respiratory syndrome target a dominant site in the S2 domain of the surface spike glycoprotein. J Virol. 2005;79(6):3401–8.
Qiu M, Shi Y, Guo Z, Chen Z, He R, Chen R, Zhou D, Dai E, Wang X, Si B, Song Y, Li J, Yang L, Wang J, Wang H, Pang X, Zhai J, Du Z, Liu Y, Zhang Y, Li L, Wang J, Sun B, Yang R. Antibody responses to individual proteins of SARS coronavirus and their neutralization activities. Microbes Infect. 2005;7(5–6):882–9.
Tang XC, Agnihothram SS, Jiao Y, Stanhope J, Graham RL, Peterson EC, Avnir Y, Tallarico AS, Sheehan J, Zhu Q, Baric RS, Marasco WA. Identification of human neutralizing antibodies against MERS-CoV and their role in virus adaptive evolution. Proc Natl Acad Sci USA. 2014;111(19):E2018-2026.
Li Y, Wan Y, Liu P, Zhao J, Lu G, Qi J, Wang Q, Lu X, Wu Y, Liu W, Zhang B, Yuen KY, Perlman S, Gao GF, Yan J. A humanized neutralizing antibody against MERS-CoV targeting the receptor-binding domain of the spike protein. Cell Res. 2015;25(11):1237–49.
Li J, Ulitzky L, Silberstein E, Taylor DR, Viscidi R. Immunogenicity and protection efficacy of monomeric and trimeric recombinant SARS coronavirus spike protein subunit vaccine candidates. Viral Immunol. 2013;26(2):126–32.
He Y, Li J, Heck S, Lustigman S, Jiang S. Antigenic and immunogenic characterization of recombinant baculovirus-expressed severe acute respiratory syndrome coronavirus spike protein: implication for vaccine design. J Virol. 2006;80(12):5757–67.
Tai W, Wang Y, Fett CA, Zhao G, Li F, Perlman S, Jiang S, Zhou Y, Du L. Recombinant receptor-binding domains of multiple Middle East respiratory syndrome coronaviruses (MERS-CoVs) induce cross-neutralizing antibodies against divergent human and camel MERS-CoVs and antibody escape mutants. J Virol. 2017. https://doi.org/10.1128/JVI.01651-16 .
Article PubMed Google Scholar
Tai W, Zhao G, Sun S, Guo Y, Wang Y, Tao X, Tseng CK, Li F, Jiang S, Du L, Zhou Y. A recombinant receptor-binding domain of MERS-CoV in trimeric form protects human dipeptidyl peptidase 4 (hDPP4) transgenic mice from MERS-CoV infection. Virology. 2016;499:375–82.
Wang Y, Tai W, Yang J, Zhao G, Sun S, Tseng CK, Jiang S, Zhou Y, Du L, Gao J. Receptor-binding domain of MERS-CoV with optimal immunogen dosage and immunization interval protects human transgenic mice from MERS-CoV infection. Hum Vaccin Immunother. 2017;13(7):1615–24.
Zhao J, Zhao J, Mangalam AK, Channappanavar R, Fett C, Meyerholz DK, Agnihothram S, Baric RS, David CS, Perlman S. Airway memory CD4(+) T cells mediate protective immunity against emerging respiratory coronaviruses. Immunity. 2016;44(6):1379–91.
He Y, Zhou Y, Siddiqui P, Niu J, Jiang S. Identification of immunodominant epitopes on the membrane protein of the severe acute respiratory syndrome-associated coronavirus. J Clin Microbiol. 2005;43(8):3718–26.
Buchholz UJ, Bukreyev A, Yang L, Lamirande EW, Murphy BR, Subbarao K, Collins PL. Contributions of the structural proteins of severe acute respiratory syndrome coronavirus to protective immunity. Proc Natl Acad Sci USA. 2004;101(26):9804–9.
Huisman W, Martina BE, Rimmelzwaan GF, Gruters RA, Osterhaus AD. Vaccine-induced enhancement of viral infections. Vaccine. 2009;27(4):505–12.
Kam YW, Kien F, Roberts A, Cheung YC, Lamirande EW, Vogel L, Chu SL, Tse J, Guarner J, Zaki SR, Subbarao K, Peiris M, Nal B, Altmeyer R. Antibodies against trimeric S glycoprotein protect hamsters against SARS-CoV challenge despite their capacity to mediate FcgammaRII-dependent entry into B cells in vitro. Vaccine. 2007;25(4):729–40.
Jaume M, Yip MS, Cheung CY, Leung HL, Li PH, Kien F, Dutry I, Callendret B, Escriou N, Altmeyer R, Nal B, Daeron M, Bruzzone R, Peiris JS. Anti-severe acute respiratory syndrome coronavirus spike antibodies trigger infection of human immune cells via a pH- and cysteine protease-independent FcgammaR pathway. J Virol. 2011;85(20):10582–97.
Wang SF, Tseng SP, Yen CH, Yang JY, Tsao CH, Shen CW, Chen KH, Liu FT, Liu WT, Chen YM, Huang JC. Antibody-dependent SARS coronavirus infection is mediated by antibodies against spike proteins. Biochem Biophys Res Commun. 2014;451(2):208–14.
Rosenthal KS, Zimmerman DH. Vaccines: all things considered. Clin Vaccine Immunol. 2006;13(8):821–9.
Bolles M, Deming D, Long K, Agnihothram S, Whitmore A, Ferris M, Funkhouser W, Gralinski L, Totura A, Heise M, Baric RS. A double-inactivated severe acute respiratory syndrome coronavirus vaccine provides incomplete protection in mice and induces increased eosinophilic proinflammatory pulmonary response upon challenge. J Virol. 2011;85(23):12201–15.
Tseng CT, Sbrana E, Iwata-Yoshikawa N, Newman PC, Garron T, Atmar RL, Peters CJ, Couch RB. Immunization with SARS coronavirus vaccines leads to pulmonary immunopathology on challenge with the SARS virus. PLoS ONE. 2012;7(4):e35421.
Iwata-Yoshikawa N, Uda A, Suzuki T, Tsunetsugu-Yokota Y, Sato Y, Morikawa S, Tashiro M, Sata T, Hasegawa H, Nagata N. Effects of Toll-like receptor stimulation on eosinophilic infiltration in lungs of BALB/c mice immunized with UV-inactivated severe acute respiratory syndrome-related coronavirus vaccine. J Virol. 2014;88(15):8597–614.
Honda-Okubo Y, Barnard D, Ong CH, Peng BH, Tseng CT, Petrovsky N. Severe acute respiratory syndrome-associated coronavirus vaccines formulated with delta inulin adjuvants provide enhanced protection while ameliorating lung eosinophilic immunopathology. J Virol. 2015;89(6):2995–3007.
He Y, Zhou Y, Liu S, Kou Z, Li W, Farzan M, Jiang S. Receptor-binding domain of SARS-CoV spike protein induces highly potent neutralizing antibodies: implication for developing subunit vaccine. Biochem Biophys Res Commun. 2004;324(2):773–81.
Du L, Zhao G, Li L, He Y, Zhou Y, Zheng BJ, Jiang S. Antigenicity and immunogenicity of SARS-CoV S protein receptor-binding domain stably expressed in CHO cells. Biochem Biophys Res Commun. 2009;384(4):486–90.
Du L, Zhao G, He Y, Guo Y, Zheng BJ, Jiang S, Zhou Y. Receptor-binding domain of SARS-CoV spike protein induces long-term protective immunity in an animal model. Vaccine. 2007;25(15):2832–8.
Du L, Zhao G, Chan CC, Sun S, Chen M, Liu Z, Guo H, He Y, Zhou Y, Zheng BJ, Jiang S. Recombinant receptor-binding domain of SARS-CoV spike protein expressed in mammalian, insect and E. coli cells elicits potent neutralizing antibody and protective immunity. Virology. 2009;393(1):144–50.
Du L, Zhao G, Chan CC, Li L, He Y, Zhou Y, Zheng BJ, Jiang S. A 219-mer CHO-expressing receptor-binding domain of SARS-CoV S protein induces potent immune responses and protective immunity. Viral Immunol. 2010;23(2):211–9.
Guo Y, Sun S, Wang K, Zhang S, Zhu W, Chen Z. Elicitation of immunity in mice after immunization with the S2 subunit of the severe acute respiratory syndrome coronavirus. DNA Cell Biol. 2005;24(8):510–5.
Liu SJ, Leng CH, Lien SP, Chi HY, Huang CY, Lin CL, Lian WC, Chen CJ, Hsieh SL, Chong P. Immunological characterizations of the nucleocapsid protein based SARS vaccine candidates. Vaccine. 2006;24(16):3100–8.
Wang L, Shi W, Joyce MG, Modjarrad K, Zhang Y, Leung K, Lees CR, Zhou T, Yassine HM, Kanekiyo M, Yang ZY, Chen X, Becker MM, Freeman M, Vogel L, Johnson JC, Olinger G, Todd JP, Bagci U, Solomon J, Mollura DJ, Hensley L, Jahrling P, Denison MR, Rao SS, Subbarao K, Kwong PD, Mascola JR, Kong WP, Graham BS. Evaluation of candidate vaccine approaches for MERS-CoV. Nat Commun. 2015;6:7712.
Adney DR, Wang L, van Doremalen N, Shi W, Zhang Y, Kong WP, Miller MR, Bushmaker T, Scott D, de Wit E, Modjarrad K, Petrovsky N, Graham BS, Bowen RA, Munster VJ. Efficacy of an adjuvanted Middle East respiratory syndrome coronavirus spike protein vaccine in dromedary camels and alpacas. Viruses. 2019;11(3):212.
Article CAS PubMed Central Google Scholar
Jiaming L, Yanfeng Y, Yao D, Yawei H, Linlin B, Baoying H, Jinghua Y, Gao GF, Chuan Q, Wenjie T. The recombinant N-terminal domain of spike proteins is a potential vaccine against Middle East respiratory syndrome coronavirus (MERS-CoV) infection. Vaccine. 2017;35(1):10–8.
Article PubMed CAS Google Scholar
Zhang N, Channappanavar R, Ma C, Wang L, Tang J, Garron T, Tao X, Tasneem S, Lu L, Tseng CT, Zhou Y, Perlman S, Jiang S, Du L. Identification of an ideal adjuvant for receptor-binding domain-based subunit vaccines against Middle East respiratory syndrome coronavirus. Cell Mol Immunol. 2016;13(2):180–90.
Lan J, Deng Y, Chen H, Lu G, Wang W, Guo X, Lu Z, Gao GF, Tan W. Tailoring subunit vaccine immunity with adjuvant combinations and delivery routes using the Middle East respiratory coronavirus (MERS-CoV) receptor-binding domain as an antigen. PLoS ONE. 2014;9(11):e112602.
Qian C, Liu X, Xu Q, Wang Z, Chen J, Li T, Zheng Q, Yu H, Gu Y, Li S, Xia N. Recent progress on the versatility of virus-like particles. Vaccines (Basel). 2020;8(1):139.
Article CAS Google Scholar
Lokugamage KG, Yoshikawa-Iwata N, Ito N, Watts DM, Wyde PR, Wang N, Newman P, Kent Tseng CT, Peters CJ, Makino S. Chimeric coronavirus-like particles carrying severe acute respiratory syndrome coronavirus (SCoV) S protein protect mice against challenge with SCoV. Vaccine. 2008;26(6):797–808.
Liu YV, Massare MJ, Barnard DL, Kort T, Nathan M, Wang L, Smith G. Chimeric severe acute respiratory syndrome coronavirus (SARS-CoV) S glycoprotein and influenza matrix 1 efficiently form virus-like particles (VLPs) that protect mice against challenge with SARS-CoV. Vaccine. 2011;29(38):6606–13.
Wang C, Zheng X, Gai W, Zhao Y, Wang H, Wang H, Feng N, Chi H, Qiu B, Li N, Wang T, Gao Y, Yang S, Xia X. MERS-CoV virus-like particles produced in insect cells induce specific humoural and cellular imminity in rhesus macaques. Oncotarget. 2017;8(8):12686–94.
Wang C, Zheng X, Gai W, Wong G, Wang H, Jin H, Feng N, Zhao Y, Zhang W, Li N, Zhao G, Li J, Yan J, Gao Y, Hu G, Yang S, Xia X. Novel chimeric virus-like particles vaccine displaying MERS-CoV receptor-binding domain induce specific humoral and cellular immune response in mice. Antiviral Res. 2017;140:55–61.
Rauch S, Jasny E, Schmidt KE, Petsch B. New vaccine technologies to combat outbreak situations. Front Immunol. 2018;9:1963.
Wang Z, Troilo PJ, Wang X, Griffiths TG, Pacchione SJ, Barnum AB, Harper LB, Pauley CJ, Niu Z, Denisova L, Follmer TT, Rizzuto G, Ciliberto G, Fattori E, Monica NL, Manam S, Ledwith BJ. Detection of integration of plasmid DNA into host genomic DNA following intramuscular injection and electroporation. Gene Ther. 2004;11(8):711–21.
Schalk JA, Mooi FR, Berbers GA, van Aerts LA, Ovelgonne H, Kimman TG. Preclinical and clinical safety studies on DNA vaccines. Hum Vaccin. 2006;2(2):45–53.
Yang ZY, Kong WP, Huang Y, Roberts A, Murphy BR, Subbarao K, Nabel GJ. A DNA vaccine induces SARS coronavirus neutralization and protective immunity in mice. Nature. 2004;428(6982):561–4.
Kim TW, Lee JH, Hung CF, Peng S, Roden R, Wang MC, Viscidi R, Tsai YC, He L, Chen PJ, Boyd DA, Wu TC. Generation and characterization of DNA vaccines targeting the nucleocapsid protein of severe acute respiratory syndrome coronavirus. J Virol. 2004;78(9):4638–45.
Zhao P, Cao J, Zhao LJ, Qin ZL, Ke JS, Pan W, Ren H, Yu JG, Qi ZT. Immune responses against SARS-coronavirus nucleocapsid protein induced by DNA vaccine. Virology. 2005;331(1):128–35.
Okada M, Okuno Y, Hashimoto S, Kita Y, Kanamaru N, Nishida Y, Tsunai Y, Inoue R, Nakatani H, Fukamizu R, Namie Y, Yamada J, Takao K, Asai R, Asaki R, Kase T, Takemoto Y, Yoshida S, Peiris JS, Chen PJ, Yamamoto N, Nomura T, Ishida I, Morikawa S, Tashiro M, Sakatani M. Development of vaccines and passive immunotherapy against SARS corona virus using SCID-PBL/hu mouse models. Vaccine. 2007;25(16):3038–40.
Wang Z, Yuan Z, Matsumoto M, Hengge UR, Chang YF. Immune responses with DNA vaccines encoded different gene fragments of severe acute respiratory syndrome coronavirus in BALB/c mice. Biochem Biophys Res Commun. 2005;327(1):130–5.
Martin JE, Louder MK, Holman LA, Gordon IJ, Enama ME, Larkin BD, Andrews CA, Vogel L, Koup RA, Roederer M, Bailer RT, Gomez PL, Nason M, Mascola JR, Nabel GJ, Graham BS, Team VRCS. A SARS DNA vaccine induces neutralizing antibody and cellular immune responses in healthy adults in a Phase I clinical trial. Vaccine. 2008;26(50):6338–43.
Zakhartchouk AN, Liu Q, Petric M, Babiuk LA. Augmentation of immune responses to SARS coronavirus by a combination of DNA and whole killed virus vaccines. Vaccine. 2005;23(35):4385–91.
Woo PC, Lau SK, Tsoi HW, Chen ZW, Wong BH, Zhang L, Chan JK, Wong LP, He W, Ma C, Chan KH, Ho DD, Yuen KY. SARS coronavirus spike polypeptide DNA vaccine priming with recombinant spike polypeptide from Escherichia coli as booster induces high titer of neutralizing antibody against SARS coronavirus. Vaccine. 2005;23(42):4959–68.
Muthumani K, Falzarano D, Reuschel EL, Tingey C, Flingai S, Villarreal DO, Wise M, Patel A, Izmirly A, Aljuaid A, Seliga AM, Soule G, Morrow M, Kraynyak KA, Khan AS, Scott DP, Feldmann F, LaCasse R, Meade-White K, Okumura A, Ugen KE, Sardesai NY, Kim JJ, Kobinger G, Feldmann H, Weiner DB. A synthetic consensus anti-spike protein DNA vaccine induces protective immunity against Middle East respiratory syndrome coronavirus in nonhuman primates. Sci Transl Med. 2015;7(301):301ra132.
Modjarrad K, Roberts CC, Mills KT, Castellano AR, Paolino K, Muthumani K, Reuschel EL, Robb ML, Racine T, Oh MD, Lamarre C, Zaidi FI, Boyer J, Kudchodkar SB, Jeong M, Darden JM, Park YK, Scott PT, Remigio C, Parikh AP, Wise MC, Patel A, Duperret EK, Kim KY, Choi H, White S, Bagarazzi M, May JM, Kane D, Lee H, Kobinger G, Michael NL, Weiner DB, Thomas SJ, Maslow JN. Safety and immunogenicity of an anti-Middle East respiratory syndrome coronavirus DNA vaccine: a phase 1, open-label, single-arm, dose-escalation trial. Lancet Infect Dis. 2019;19(9):1013–22.
Smith TRF, Patel A, Ramos S, Elwood D, Zhu X, Yan J, Gary EN, Walker SN, Schultheis K, Purwar M, Xu Z, Walters J, Bhojnagarwala P, Yang M, Chokkalingam N, Pezzoli P, Parzych E, Reuschel EL, Doan A, Tursi N, Vasquez M, Choi J, Tello-Ruiz E, Maricic I, Bah MA, Wu Y, Amante D, Park DH, Dia Y, Ali AR, Zaidi FI, Generotti A, Kim KY, Herring TA, Reeder S, Andrade VM, Buttigieg K, Zhao G, Wu JM, Li D, Bao L, Liu J, Deng W, Qin C, Brown AS, Khoshnejad M, Wang N, Chu J, Wrapp D, McLellan JS, Muthumani K, Wang B, Carroll MW, Kim JJ, Boyer J, Kulp DW, Humeau L, Weiner DB, Broderick KE. Immunogenicity of a DNA vaccine candidate for COVID-19. Nat Commun. 2020;11(1):2601.
Al-Amri SS, Abbas AT, Siddiq LA, Alghamdi A, Sanki MA, Al-Muhanna MK, Alhabbab RY, Azhar EI, Li X, Hashem AM. Immunogenicity of Candidate MERS-CoV DNA vaccines based on the spike protein. Sci Rep. 2017;7:44875.
Fausther-Bovendo H, Kobinger GP. Pre-existing immunity against Ad vectors: humoral, cellular, and innate response, what’s important? Hum Vaccin Immunother. 2014;10(10):2875–84.
Knuchel MC, Marty RR, Morin TN, Ilter O, Zuniga A, Naim HY. Relevance of a pre-existing measles immunity prior immunization with a recombinant measles virus vector. Hum Vacc Immunother. 2013;9(3):599–606.
Enjuanes L, Dediego ML, Alvarez E, Deming D, Sheahan T, Baric R. Vaccines to prevent severe acute respiratory syndrome coronavirus-induced disease. Virus Res. 2008;133(1):45–62.
Schindewolf C, Menachery VD. Middle East respiratory syndrome vaccine candidates: cautious optimism. Viruses. 2019;11(1):74.
Gao W, Tamin A, Soloff A, D’Aiuto L, Nwanegbo E, Robbins PD, Bellini WJ, Barratt-Boyes S, Gambotto A. Effects of a SARS-associated coronavirus vaccine in monkeys. Lancet. 2003;362(9399):1895–6.
Liu RY, Wu LZ, Huang BJ, Huang JL, Zhang YL, Ke ML, Wang JM, Tan WP, Zhang RH, Chen HK, Zeng YX, Huang W. Adenoviral expression of a truncated S1 subunit of SARS-CoV spike protein results in specific humoral immune responses against SARS-CoV in rats. Virus Res. 2005;112(1–2):24–31.
See RH, Petric M, Lawrence DJ, Mok CPY, Rowe T, Zitzow LA, Karunakaran KP, Voss TG, Brunham RC, Gauldie J, Finlay BB, Roper RL. Severe acute respiratory syndrome vaccine efficacy in ferrets: whole killed virus and adenovirus-vectored vaccines. J Gen Virol. 2008;89(Pt 9):2136–46.
Kobinger GP, Figueredo JM, Rowe T, Zhi Y, Gao G, Sanmiguel JC, Bell P, Wivel NA, Zitzow LA, Flieder DB, Hogan RJ, Wilson JM. Adenovirus-based vaccine prevents pneumonia in ferrets challenged with the SARS coronavirus and stimulates robust immune responses in macaques. Vaccine. 2007;25(28):5220–31.
Volz A, Sutter G. Modified vaccinia virus Ankara: history, value in basic research, and current perspectives for vaccine development. Adv Virus Res. 2017;97:187–243.
Bisht H, Roberts A, Vogel L, Bukreyev A, Collins PL, Murphy BR, Subbarao K, Moss B. Severe acute respiratory syndrome coronavirus spike protein expressed by attenuated vaccinia virus protectively immunizes mice. Proc Natl Acad Sci USA. 2004;101(17):6641–6.
Chen Z, Zhang L, Qin C, Ba L, Yi CE, Zhang F, Wei Q, He T, Yu W, Yu J, Gao H, Tu X, Gettie A, Farzan M, Yuen KY, Ho DD. Recombinant modified vaccinia virus Ankara expressing the spike glycoprotein of severe acute respiratory syndrome coronavirus induces protective neutralizing antibodies primarily targeting the receptor binding region. J Virol. 2005;79(5):2678–88.
Czub M, Weingartl H, Czub S, He R, Cao J. Evaluation of modified vaccinia virus Ankara based recombinant SARS vaccine in ferrets. Vaccine. 2005;23(17–18):2273–9.
Weingartl H, Czub M, Czub S, Neufeld J, Marszal P, Gren J, Smith G, Jones S, Proulx R, Deschambault Y, Grudeski E, Andonov A, He R, Li Y, Copps J, Grolla A, Dick D, Berry J, Ganske S, Manning L, Cao J. Immunization with modified vaccinia virus Ankara-based recombinant vaccine against severe acute respiratory syndrome is associated with enhanced hepatitis in ferrets. J Virol. 2004;78(22):12672–6.
Deming D, Sheahan T, Heise M, Yount B, Davis N, Sims A, Suthar M, Harkema J, Whitmore A, Pickles R, West A, Donaldson E, Curtis K, Johnston R, Baric R. Vaccine efficacy in senescent mice challenged with recombinant SARS-CoV bearing epidemic and zoonotic spike variants. PLoS Med. 2006;3(12):e525.
Sheahan T, Whitmore A, Long K, Ferris M, Rockx B, Funkhouser W, Donaldson E, Gralinski L, Collier M, Heise M, Davis N, Johnston R, Baric RS. Successful vaccination strategies that protect aged mice from lethal challenge from influenza virus and heterologous severe acute respiratory syndrome coronavirus. J Virol. 2011;85(1):217–30.
Bukreyev A, Lamirande EW, Buchholz UJ, Vogel LN, Elkins WR, St Claire M, Murphy BR, Subbarao K, Collins PL. Mucosal immunisation of African green monkeys ( Cercopithecus aethiops ) with an attenuated parainfluenza virus expressing the SARS coronavirus spike protein for the prevention of SARS. Lancet. 2004;363(9427):2122–7.
Kapadia SU, Rose JK, Lamirande E, Vogel L, Subbarao K, Roberts A. Long-term protection from SARS coronavirus infection conferred by a single immunization with an attenuated VSV-based vaccine. Virology. 2005;340(2):174–82.
Kim E, Okada K, Kenniston T, Raj VS, AlHajri MM, Farag EA, AlHajri F, Osterhaus AD, Haagmans BL, Gambotto A. Immunogenicity of an adenoviral-based Middle East Respiratory Syndrome coronavirus vaccine in BALB/c mice. Vaccine. 2014;32(45):5975–82.
Guo X, Deng Y, Chen H, Lan J, Wang W, Zou X, Hung T, Lu Z, Tan W. Systemic and mucosal immunity in mice elicited by a single immunization with human adenovirus type 5 or 41 vector-based vaccines carrying the spike protein of Middle East respiratory syndrome coronavirus. Immunology. 2015;145(4):476–84.
Jung SY, Kang KW, Lee EY, Seo DW, Kim HL, Kim H, Kwon T, Park HL, Kim H, Lee SM, Nam JH. Heterologous prime-boost vaccination with adenoviral vector and protein nanoparticles induces both Th1 and Th2 responses against Middle East respiratory syndrome coronavirus. Vaccine. 2018;36(24):3468–76.
Zhu FC, Li YH, Guan XH, Hou LH, Wang WJ, Li JX, Wu SP, Wang BS, Wang Z, Wang L, Jia SY, Jiang HD, Wang L, Jiang T, Hu Y, Gou JB, Xu SB, Xu JJ, Wang XW, Wang W, Chen W. Safety, tolerability, and immunogenicity of a recombinant adenovirus type-5 vectored COVID-19 vaccine: a dose-escalation, open-label, non-randomised, first-in-human trial. Lancet. 2020;395(10240):1845–54.
Zhu FC, Guan XH, Li YH, Huang JY, Jiang T, Hou LH, Li JX, Yang BF, Wang L, Wang WJ, Wu SP, Wang Z, Wu XH, Xu JJ, Zhang Z, Jia SY, Wang BS, Hu Y, Liu JJ, Zhang J, Qian XA, Li Q, Pan HX, Jiang HD, Deng P, Gou JB, Wang XW, Wang XH, Chen W. Immunogenicity and safety of a recombinant adenovirus type-5-vectored COVID-19 vaccine in healthy adults aged 18 years or older: a randomised, double-blind, placebo-controlled, phase 2 trial. Lancet. 2020;396(10249):479–88.
Alharbi NK, Padron-Regalado E, Thompson CP, Kupke A, Wells D, Sloan MA, Grehan K, Temperton N, Lambe T, Warimwe G, Becker S, Hill AVS, Gilbert SC. ChAdOx1 and MVA based vaccine candidates against MERS-CoV elicit neutralising antibodies and cellular immune responses in mice. Vaccine. 2017;35(30):3780–8.
Munster VJ, Wells D, Lambe T, Wright D, Fischer RJ, Bushmaker T, Saturday G, van Doremalen N, Gilbert SC, de Wit E, Warimwe GM. Protective efficacy of a novel simian adenovirus vaccine against lethal MERS-CoV challenge in a transgenic human DPP4 mouse model. NPJ Vaccines. 2017;2:28.
Alharbi NK, Qasim I, Almasoud A, Aljami HA, Alenazi MW, Alhafufi A, Aldibasi OS, Hashem AM, Kasem S, Albrahim R, Aldubaib M, Almansour A, Temperton NJ, Kupke A, Becker S, Abu-Obaidah A, Alkarar A, Yoon IK, Azhar E, Lambe T, Bayoumi F, Aldowerij A, Ibrahim OH, Gilbert SC, Balkhy HH. Humoral immunogenicity and efficacy of a single dose of ChAdOx1 MERS vaccine candidate in dromedary camels. Sci Rep. 2019;9(1):16292.
van Doremalen N, Haddock E, Feldmann F, Meade-White K, Bushmaker T, Fischer RJ, Okumura A, Hanley PW, Saturday G, Edwards NJ, Clark MHA, Lambe T, Gilbert SC, Munster VJ. A single dose of ChAdOx1 MERS provides protective immunity in rhesus macaques. Sci Adv. 2020;6(24):eaba8399.
Folegatti PM, Bittaye M, Flaxman A, Lopez FR, Bellamy D, Kupke A, Mair C, Makinson R, Sheridan J, Rohde C, Halwe S, Jeong Y, Park YS, Kim JO, Song M, Boyd A, Tran N, Silman D, Poulton I, Datoo M, Marshall J, Themistocleous Y, Lawrie A, Roberts R, Berrie E, Becker S, Lambe T, Hill A, Ewer K, Gilbert S. Safety and immunogenicity of a candidate Middle East respiratory syndrome coronavirus viral-vectored vaccine: a dose-escalation, open-label, non-randomised, uncontrolled, phase 1 trial. Lancet Infect Dis. 2020;20(7):816–26.
Folegatti PM, Ewer KJ, Aley PK, Angus B, Becker S, Belij-Rammerstorfer S, Bellamy D, Bibi S, Bittaye M, Clutterbuck EA, Dold C, Faust SN, Finn A, Flaxman AL, Hallis B, Heath P, Jenkin D, Lazarus R, Makinson R, Minassian AM, Pollock KM, Ramasamy M, Robinson H, Snape M, Tarrant R, Voysey M, Green C, Douglas AD, Hill AVS, Lambe T, Gilbert SC, Pollard AJ, Oxford CVTG. Safety and immunogenicity of the ChAdOx1 nCoV-19 vaccine against SARS-CoV-2: a preliminary report of a phase 1/2, single-blind, randomised controlled trial. Lancet. 2020;396(10249):467–78.
Volz A, Kupke A, Song F, Jany S, Fux R, Shams-Eldin H, Schmidt J, Becker C, Eickmann M, Becker S, Sutter G. Protective efficacy of recombinant modified vaccinia virus Ankara delivering Middle East respiratory syndrome coronavirus spike glycoprotein. J Virol. 2015;89(16):8651–6.
Haagmans BL, van den Brand JM, Raj VS, Volz A, Wohlsein P, Smits SL, Schipper D, Bestebroer TM, Okba N, Fux R, Bensaid A, Solanes Foz D, Kuiken T, Baumgartner W, Segales J, Sutter G, Osterhaus AD. An orthopoxvirus-based vaccine reduces virus excretion after MERS-CoV infection in dromedary camels. Science. 2016;351(6268):77–81.
Koch T, Dahlke C, Fathi A, Kupke A, Krahling V, Okba NMA, Halwe S, Rohde C, Eickmann M, Volz A, Hesterkamp T, Jambrecina A, Borregaard S, Ly ML, Zinser ME, Bartels E, Poetsch JSH, Neumann R, Fux R, Schmiedel S, Lohse AW, Haagmans BL, Sutter G, Becker S, Addo MM. Safety and immunogenicity of a modified vaccinia virus Ankara vector vaccine candidate for Middle East respiratory syndrome: an open-label, phase 1 trial. Lancet Infect Dis. 2020. https://doi.org/10.1016/S1473-3099(20)30248-6 .
Agnihothram S, Gopal R, Yount BL Jr, Donaldson EF, Menachery VD, Graham RL, Scobey TD, Gralinski LE, Denison MR, Zambon M, Baric RS. Evaluation of serologic and antigenic relationships between middle eastern respiratory syndrome coronavirus and other coronaviruses to develop vaccine platforms for the rapid response to emerging coronaviruses. J Infect Dis. 2014;209(7):995–1006.
Malczyk AH, Kupke A, Prufer S, Scheuplein VA, Hutzler S, Kreuz D, Beissert T, Bauer S, Hubich-Rau S, Tondera C, Eldin HS, Schmidt J, Vergara-Alert J, Suzer Y, Seifried J, Hanschmann KM, Kalinke U, Herold S, Sahin U, Cichutek K, Waibler Z, Eickmann M, Becker S, Muhlebach MD. A highly immunogenic and protective Middle East respiratory syndrome coronavirus vaccine based on a recombinant measles virus vaccine platform. J Virol. 2015;89(22):11654–67.
Wirblich C, Coleman CM, Kurup D, Abraham TS, Bernbaum JG, Jahrling PB, Hensley LE, Johnson RF, Frieman MB, Schnell MJ. One-health: a safe, efficient, dual-use vaccine for humans and animals against Middle East respiratory syndrome coronavirus and rabies virus. J Virol. 2017;91(2):e02040-16.
Liu RQ, Ge JY, Wang JL, Shao Y, Zhang HL, Wang JL, Wen ZY, Bu ZG. Newcastle disease virus-based MERS-CoV candidate vaccine elicits high-level and lasting neutralizing antibodies in Bactrian camels. J Integr Agric. 2017;16(10):2264–73.
Liu R, Wang J, Shao Y, Wang X, Zhang H, Shuai L, Ge J, Wen Z, Bu Z. A recombinant VSV-vectored MERS-CoV vaccine induces neutralizing antibody and T cell responses in rhesus monkeys after single dose immunization. Antiviral Res. 2018;150:30–8.
Takasuka N, Fujii H, Takahashi Y, Kasai M, Morikawa S, Itamura S, Ishii K, Sakaguchi M, Ohnishi K, Ohshima M, Hashimoto S, Odagiri T, Tashiro M, Yoshikura H, Takemori T, Tsunetsugu-Yokota Y. A subcutaneously injected UV-inactivated SARS coronavirus vaccine elicits systemic humoral immunity in mice. Int Immunol. 2004;16(10):1423–30.
Qu D, Zheng B, Yao X, Guan Y, Yuan ZH, Zhong NS, Lu LW, Xie JP, Wen YM. Intranasal immunization with inactivated SARS-CoV (SARS-associated coronavirus) induced local and serum antibodies in mice. Vaccine. 2005;23(7):924–31.
Lin JT, Zhang JS, Su N, Xu JG, Wang N, Chen JT, Chen X, Liu YX, Gao H, Jia YP, Liu Y, Sun RH, Wang X, Yu DZ, Hai R, Gao Q, Ning Y, Wang HX, Li MC, Kan B, Dong GM, An Q, Wang YQ, Han J, Qin C, Yin WD, Dongs XP. Safety and immunogenicity from a phase I trial of inactivated severe acute respiratory syndrome coronavirus vaccine. Antivir Ther. 2007;12(7):1107–13.
CAS PubMed Google Scholar
Agrawal AS, Tao X, Algaissi A, Garron T, Narayanan K, Peng BH, Couch RB, Tseng CT. Immunization with inactivated Middle East Respiratory Syndrome coronavirus vaccine leads to lung immunopathology on challenge with live virus. Hum Vaccin Immunother. 2016;12(9):2351–6.
Deng Y, Lan J, Bao L, Huang B, Ye F, Chen Y, Yao Y, Wang W, Qin C, Tan W. Enhanced protection in mice induced by immunization with inactivated whole viruses compare to spike protein of middle east respiratory syndrome coronavirus. Emerg Microbes Infect. 2018;7(1):60.
Minor PD. Live attenuated vaccines: historical successes and current challenges. Virology. 2015;479–480:379–92.
Lamirande EW, DeDiego ML, Roberts A, Jackson JP, Alvarez E, Sheahan T, Shieh WJ, Zaki SR, Baric R, Enjuanes L, Subbarao K. A live attenuated severe acute respiratory syndrome coronavirus is immunogenic and efficacious in golden Syrian hamsters. J Virol. 2008;82(15):7721–4.
Menachery VD, Gralinski LE, Mitchell HD, Dinnon KH 3rd, Leist SR, Yount BL Jr, McAnarney ET, Graham RL, Waters KM, Baric RS. Combination attenuation offers strategy for live attenuated coronavirus vaccines. J Virol. 2018;92(17):e00710-e718.
Menachery VD, Gralinski LE, Mitchell HD, Dinnon KH 3rd, Leist SR, Yount BL Jr, Graham RL, McAnarney ET, Stratton KG, Cockrell AS, Debbink K, Sims AC, Waters KM, Baric RS. Middle East Respiratory syndrome coronavirus nonstructural protein 16 is necessary for interferon resistance and viral pathogenesis. mSphere. 2017;2(6):e00346-17.
Graham RL, Becker MM, Eckerle LD, Bolles M, Denison MR, Baric RS. A live, impaired-fidelity coronavirus vaccine protects in an aged, immunocompromised mouse model of lethal disease. Nat Med. 2012;18(12):1820–6.
Schoeman D, Fielding BC. Coronavirus envelope protein: current knowledge. Virol J. 2019;16(1):69.
DeDiego ML, Nieto-Torres JL, Jimenez-Guardeno JM, Regla-Nava JA, Castano-Rodriguez C, Fernandez-Delgado R, Usera F, Enjuanes L. Coronavirus virulence genes with main focus on SARS-CoV envelope gene. Virus Res. 2014;194:124–37.
Menachery VD, Debbink K, Baric RS. Coronavirus non-structural protein 16: evasion, attenuation, and possible treatments. Virus Res. 2014;194:191–9.
Robson F, Khan KS, Le TK, Paris C, Demirbag S, Barfuss P, Rocchi P, Ng WL. Coronavirus RNA proofreading: molecular basis and therapeutic targeting. Mol Cell. 2020;79(5):710–27.
van Riel D, de Wit E. Next-generation vaccine platforms for COVID-19. Nat Mater. 2020;19(8):810–2.
Lurie N, Saville M, Hatchett R, Halton J. Developing covid-19 vaccines at pandemic speed. N Engl J Med. 2020;382(21):1969–73.
Johnson-&-Johnson. Johnson & Johnson announces a lead vaccine candidate for COVID-19; landmark new partnership with U.S. Department of Health & Human Services; and commitment to supply one billion vaccines worldwide for emergency pandemic use. 2020.
GSK. GSK announces intention to produce 1 billion doses of pandemic vaccine adjuvant in 2021 to support multiple COVID-19 vaccine collaborations. 2020.
Chemical-&-Engineering-News. Moderna picks Lonza to make 1 billion doses of its coronavirus vaccine. 2020.
CNN-Health. US taxpayers are funding six Covid vaccines. Here's how they work. 2020.
REUTERS. EU to use $2.7 billion fund to buy promising COVID-19 vaccines. 2020.
AP-News. China aims to make 1 billion COVID-19 vaccine doses a year. 2020.
World-Health-Organization. Draft landscape of COVID-19 candidate vaccines. Geneva: World-Health-Organization; 2020c.
Novavax. NVX-CoV2373 COVID-19 Vaccine candidate phase 1/2, part 1, clinical trial results. 2020.
Keech C, Albert G, Cho I, Robertson A, Reed P, Neal S, Plested JS, Zhu M, Cloney-Clark S, Zhou H, Smith G, Patel N, Frieman MB, Haupt RE, Logue J, McGrath M, Weston S, Piedra PA, Desai C, Callahan K, Lewis M, Price-Abbott P, Formica N, Shinde V, Fries L, Lickliter JD, Griffin P, Wilkinson B, Glenn GM. Phase 1–2 trial of a SARS-CoV-2 recombinant spike protein nanoparticle vaccine. N Engl J Med. 2020. https://doi.org/10.1056/NEJMoa2026920 .
Dai L, Zheng T, Xu K, Han Y, Xu L, Huang E, An Y, Cheng Y, Li S, Liu M, Yang M, Li Y, Cheng H, Yuan Y, Zhang W, Ke C, Wong G, Qi J, Qin C, Yan J, Gao GF. A universal design of betacoronavirus vaccines against COVID-19, MERS, and SARS. Cell. 2020;182(3):722-733 e711.
Diehl MC, Lee JC, Daniels SE, Tebas P, Khan AS, Giffear M, Sardesai NY, Bagarazzi ML. Tolerability of intramuscular and intradermal delivery by CELLECTRA((R)) adaptive constant current electroporation device in healthy volunteers. Hum Vaccin Immunother. 2013;9(10):2246–52.
Patel A, Walters J, Reuschel EL, Schultheis K, Parzych E, Gary EN, Maricic I, Purwar M, Eblimit Z, Walker SN, Guimet D, Bhojnagarwala P, Doan A, Xu Z, Elwood D, Reeder SM, Pessaint L, Kim KY, Cook A, Chokkalingam N, Finneyfrock B, Tello-Ruiz E, Dodson A, Choi J, Generotti A, Harrison J, Tursi NJ, Andrade VM, Dia Y, Zaidi FI, Andersen H, Lewis MG, Muthumani K, Kim JJ, Kulp DW, Humeau LM, Ramos S, Smith TRF, Weiner DB, Broderick KE. Intradermal-delivered DNA vaccine provides anamnestic protection in a rhesus macaque SARS-CoV-2 challenge model. bioRxiv. 2020. https://doi.org/10.1101/2020.07.28.225649 .
INOVIO-Pharmaceuticals. INOVIO announces positive interim phase 1 data for INO-4800 vaccine for COVID-19. 2020. http://ir.inovio.com/news-releases/news-releases-details/2020/INOVIO-Announces-Positive-Interim-Phase-1-Data-For-INO-4800-Vaccine-for-COVID-19/default.aspx .
Kauffman KJ, Webber MJ, Anderson DG. Materials for non-viral intracellular delivery of messenger RNA therapeutics. J Control Release. 2016;240:227–34.
Sahin U, Kariko K, Tureci O. mRNA-based therapeutics–developing a new class of drugs. Nat Rev Drug Discov. 2014;13(10):759–80.
Pardi N, Hogan MJ, Porter FW, Weissman D. mRNA vaccines—a new era in vaccinology. Nat Rev Drug Discov. 2018;17(4):261–79.
Jackson LA, Anderson EJ, Rouphael NG, Roberts PC, Makhene M, Coler RN, McCullough MP, Chappell JD, Denison MR, Stevens LJ, Pruijssers AJ, McDermott A, Flach B, Doria-Rose NA, Corbett KS, Morabito KM, O’Dell S, Schmidt SD, Swanson 2nd PA, Padilla M, Mascola JR, Neuzil KM, Bennett H, Sun W, Peters E, Makowski M, Albert J, Cross K, Buchanan W, Pikaart-Tautges R, Ledgerwood JE, Graham BS, Beigel JH, m RNASG. An mRNA vaccine against SARS-CoV-2—preliminary report. N Engl J Med. 2020. https://doi.org/10.1056/NEJMoa2022483 .
Article Google Scholar
Anderson EJ, Rouphael NG, Widge AT, Jackson LA, Roberts PC, Makhene M, Chappell JD, Denison MR, Stevens LJ, Pruijssers AJ, McDermott AB, Flach B, Lin BC, Doria-Rose NA, O'Dell S, Schmidt SD, Corbett KS, Swanson 2nd PA, Padilla M, Neuzil KM, Bennett H, Leav B, Makowski M, Albert J, Cross K, Edara VV, Floyd K, Suthar MS, Martinez DR, Baric R, Buchanan W, Luke CJ, Phadke VK, Rostad CA, Ledgerwood JE, Graham BS, Beigel JH, m RNASG. Safety and immunogenicity of SARS-CoV-2 mRNA-1273 vaccine in older adults. N Engl J Med. 2020. https://doi.org/10.1056/NEJMoa2028436 .
Moderna’s COVID-19 Vaccine Candidate Meets its Primary Efficacy Endpoint in the First Interim Analysis of the Phase 3 COVE Study. Moderna , 2020. https://investors.modernatx.com/news-releases/news-release-details/modernas-covid-19-vaccine-candidate-meets-its-primary-efficacy .
Genetic-Engineering-&-Biotechnology-News. BioNTech, Pfizer, and Fosun Pharma—BNT162. Genetic Engineering & Biotechnology News. 2020.
Mulligan MJ, Lyke KE, Kitchin N, Absalon J, Gurtman A, Lockhart S, Neuzil K, Raabe V, Bailey R, Swanson KA, Li P, Koury K, Kalina W, Cooper D, Fontes-Garfias C, Shi PY, Tureci O, Tompkins KR, Walsh EE, Frenck R, Falsey AR, Dormitzer PR, Gruber WC, Sahin U, Jansen KU. Phase 1/2 study of COVID-19 RNA vaccine BNT162b1 in adults. Nature. 2020. https://doi.org/10.1038/s41586-020-2639-4 .
Sahin U, Muik A, Derhovanessian E, Vogler I, Kranz LM, Vormehr M, Baum A, Pascal K, Quandt J, Maurus D, Brachtendorf S, Lorks V, Sikorski J, Hilker R, Becker D, Eller AK, Grutzner J, Boesler C, Rosenbaum C, Kuhnle MC, Luxemburger U, Kemmer-Bruck A, Langer D, Bexon M, Bolte S, Kariko K, Palanche T, Fischer B, Schultz A, Shi PY, Fontes-Garfias C, Perez JL, Swanson KA, Loschko J, Scully IL, Cutler M, Kalina W, Kyratsous CA, Cooper D, Dormitzer PR, Jansen KU, Tureci O. COVID-19 vaccine BNT162b1 elicits human antibody and TH1 T-cell responses. Nature. 2020. https://doi.org/10.1038/s41586-020-2814-7 .
Walsh EE, Frenck RW Jr, Falsey AR, Kitchin N, Absalon J, Gurtman A, Lockhart S, Neuzil K, Mulligan MJ, Bailey R, Swanson KA, Li P, Koury K, Kalina W, Cooper D, Fontes-Garfias C, Shi PY, Tureci O, Tompkins KR, Lyke KE, Raabe V, Dormitzer PR, Jansen KU, Sahin U, Gruber WC. Safety and immunogenicity of two RNA-based covid-19 vaccine candidates. N Engl J Med. 2020. https://doi.org/10.1056/nejmoa2027906 .
Pfizer and BioNTech Conclude Phase 3 Study of COVID-19 Vaccine Candidate, Meeting All Primary Efficacy Endpoints. Pfizer , 2020. https://www.pfizer.com/news/press-release/press-release-detail/pfizer-and-biontech-conclude-phase-3-study-covid-19-vaccine .
The-New-York-Times. AstraZeneca Pauses Vaccine Trial for Safety Review. 2020.
Astrazeneca. COVID-19 vaccine AZD1222 clinical trials resumed in the UK. 2020.
AZD1222 vaccine met primary efficacy endpoint in preventing COVID-19. Astrazeneca , 2020. https://www.astrazeneca.com/media-centre/press-releases/2020/azd1222hlr.html .
Logunov DY, Dolzhikova IV, Zubkova OV, Tukhvatullin AI, Shcheblyakov DV, Dzharullaeva AS, Grousova DM, Erokhova AS, Kovyrshina AV, Botikov AG, Izhaeva FM, Popova O, Ozharovskaya TA, Esmagambetov IB, Favorskaya IA, Zrelkin DI, Voronina DV, Shcherbinin DN, Semikhin AS, Simakova YV, Tokarskaya EA, Lubenets NL, Egorova DA, Shmarov MM, Nikitenko NA, Morozova LF, Smolyarchuk EA, Kryukov EV, Babira VF, Borisevich SV, Naroditsky BS, Gintsburg AL. Safety and immunogenicity of an rAd26 and rAd5 vector-based heterologous prime-boost COVID-19 vaccine in two formulations: two open, non-randomised phase 1/2 studies from Russia. Lancet. 2020;396(10255):887–97.
Second Interim Analysis of Clinical Trial Data Showed a 91.4% Efficacy for the Sputnik V Vaccine on Day 28 After the First Dose; Vaccine Efficacy is Over 95% 42 Days After the First Dose. Sputnik V , 2020. https://sputnikvaccine.com/newsroom/pressreleases/second-interim-analysis-of-clinical-trial-data-showed-a-91-4-efficacy-for-the-sputnik-v-vaccine-on-d/ .
Gao Q, Bao L, Mao H, Wang L, Xu K, Yang M, Li Y, Zhu L, Wang N, Lv Z, Gao H, Ge X, Kan B, Hu Y, Liu J, Cai F, Jiang D, Yin Y, Qin C, Li J, Gong X, Lou X, Shi W, Wu D, Zhang H, Zhu L, Deng W, Li Y, Lu J, Li C, Wang X, Yin W, Zhang Y, Qin C. Development of an inactivated vaccine candidate for SARS-CoV-2. Science. 2020;369(6499):77–81.
Xia S, Duan K, Zhang Y, Zhao D, Zhang H, Xie Z, Li X, Peng C, Zhang Y, Zhang W, Yang Y, Chen W, Gao X, You W, Wang X, Wang Z, Shi Z, Wang Y, Yang X, Zhang L, Huang L, Wang Q, Lu J, Yang Y, Guo J, Zhou W, Wan X, Wu C, Wang W, Huang S, Du J, Meng Z, Pan A, Yuan Z, Shen S, Guo W, Yang X. Effect of an inactivated vaccine against SARS-CoV-2 on safety and immunogenicity outcomes: interim analysis of 2 randomized clinical trials. JAMA. 2020;324(10):951–60.
Wang H, Zhang Y, Huang B, Deng W, Quan Y, Wang W, Xu W, Zhao Y, Li N, Zhang J, Liang H, Bao L, Xu Y, Ding L, Zhou W, Gao H, Liu J, Niu P, Zhao L, Zhen W, Fu H, Yu S, Zhang Z, Xu G, Li C, Lou Z, Xu M, Qin C, Wu G, Gao GF, Tan W, Yang X. Development of an inactivated vaccine candidate, BBIBP-CorV, with potent protection against SARS-CoV-2. Cell. 2020;182(3):713-721 e719.
Xia S, Zhang Y, Wang Y, Wang H, Yang Y, Gao GF, Tan W, Wu G, Xu M, Lou Z, Huang W, Xu W, Huang B, Wang H, Wang W, Zhang W, Li N, Xie Z, Ding L, You W, Zhao Y, Yang X, Liu Y, Wang Q, Huang L, Yang Y, Xu G, Luo B, Wang W, Liu P, Guo W, Yang X. Safety and immunogenicity of an inactivated SARS-CoV-2 vaccine, BBIBP-CorV: a randomised, double-blind, placebo-controlled, phase 1/2 trial. Lancet Infect Dis. 2020. https://doi.org/10.1016/S1473-3099(20)30831-8 .
AIVITA-Biomedical. SARS-COV-2 VACCINE. 2020.
Zhou LK, Zhou Z, Jiang XM, Zheng Y, Chen X, Fu Z, Xiao G, Zhang CY, Zhang LK, Yi Y. Absorbed plant MIR2911 in honeysuckle decoction inhibits SARS-CoV-2 replication and accelerates the negative conversion of infected patients. Cell Discov. 2020;6:54.
Stensballe LG, Nante E, Jensen IP, Kofoed PE, Poulsen A, Jensen H, Newport M, Marchant A, Aaby P. Acute lower respiratory tract infections and respiratory syncytial virus in infants in Guinea-Bissau: a beneficial effect of BCG vaccination for girls community based case-control study. Vaccine. 2005;23(10):1251–7.
Spencer JC, Ganguly R, Waldman RH. Nonspecific protection of mice against influenza virus infection by local or systemic immunization with Bacille Calmette-Guerin. J Infect Dis. 1977;136(2):171–5.
Starr SE, Visintine AM, Tomeh MO, Nahmias AJ. Effects of immunostimulants on resistance of newborn mice to herpes simplex type 2 infection. Proc Soc Exp Biol Med. 1976;152(1):57–60.
O’Neill LAJ, Netea MG. BCG-induced trained immunity: can it offer protection against COVID-19? Nat Rev Immunol. 2020;20(6):335–7.
Miller A, Reandelar MJ, Fasciglione K, Roumenova V, Li Y, Otazu GH. Correlation between universal BCG vaccination policy and reduced mortality for COVID-19. medRxiv. 2020. https://doi.org/10.1101/2020.03.24.20042937 .
Cao WC, Liu W, Zhang PH, Zhang F, Richardus JH. Disappearance of antibodies to SARS-associated coronavirus after recovery. N Engl J Med. 2007;357(11):1162–3.
Wu LP, Wang NC, Chang YH, Tian XY, Na DY, Zhang LY, Zheng L, Lan T, Wang LF, Liang GD. Duration of antibody responses after severe acute respiratory syndrome. Emerg Infect Dis. 2007;13(10):1562–4.
Iwasaki A. What reinfections mean for COVID-19. Lancet Infect Dis. 2020. https://doi.org/10.1016/S1473-3099(20)30783-0 .
Ko JH, Joo EJ, Park SJ, Baek JY, Kim WD, Jee J, Kim CJ, Jeong C, Kim YJ, Shon HJ, Kang ES, Choi YK, Peck KR. Neutralizing antibody production in asymptomatic and mild COVID-19 patients, in comparison with pneumonic COVID-19 patients. J Clin Med. 2020;9(7):2268.
Callaway E. The coronavirus is mutating—does it matter? Nature. 2020;585(7824):174–7.
Korber B, Fischer WM, Gnanakaran S, Yoon H, Theiler J, Abfalterer W, Hengartner N, Giorgi EE, Bhattacharya T, Foley B, Hastie KM, Parker MD, Partridge DG, Evans CM, Freeman TM, de Silva TI, Sheffield C-GG, McDanal C, Perez LG, Tang H, Moon-Walker A, Whelan SP, LaBranche CC, Saphire EO, Montefiori DC. Tracking changes in SARS-CoV-2 spike: evidence that D614G increases infectivity of the COVID-19 virus. Cell. 2020;182(4):812-827 e819.
Zhang L, Jackson CB, Mou H, Ojha A, Rangarajan ES, Izard T, Farzan M, Choe H. The D614G mutation in the SARS-CoV-2 spike protein reduces S1 shedding and increases infectivity. bioRxiv. 2020. https://doi.org/10.1101/2020.06.12.148726 .
Beigel JH, Tomashek KM, Dodd LE, Mehta AK, Zingman BS, Kalil AC, Hohmann E, Chu HY, Luetkemeyer A, Kline S, Lopez de Castilla D, Finberg RW, Dierberg K, Tapson V, Hsieh L, Patterson TF, Paredes R, Sweeney DA, Short WR, Touloumi G, Lye DC, Ohmagari N, Oh MD, Ruiz-Palacios GM, Benfield T, Fatkenheuer G, Kortepeter MG, Atmar RL, Creech CB, Lundgren J, Babiker AG, Pett S, Neaton JD, Burgess TH, Bonnett T, Green M, Makowski M, Osinusi A, Nayak S, Lane HC, Members A-SG. Remdesivir for the treatment of covid-19—final report. N Engl J Med. 2020. https://doi.org/10.1056/NEJMoa2007764
Group R.C., Horby P., Lim W.S., Emberson J.R., Mafham M., Bell J.L., Linsell L., Staplin N., Brightling C., Ustianowski A., Elmahi E., Prudon B., Green C., Felton T., Chadwick D., Rege K., Fegan C., Chappell L.C., Faust S.N., Jaki T., Jeffery K., Montgomery A., Rowan K., Juszczak E., Baillie J.K., Haynes R., Landray M.J. Dexamethasone in hospitalized patients with covid-19—preliminary report. N Engl J Med. 2020. https://doi.org/10.1056/NEJMoa2021436 .
Download references
Acknowledgements
Author information.
Yen-Der Li and Wei-Yu Chi have contributed equally to this work
Authors and Affiliations
Department of Molecular and Cellular Biology, Harvard University, Cambridge, MA, USA
Yen-Der Li & Jun-Han Su
Department of Pathology, School of Medicine, Johns Hopkins University, Baltimore, MD, USA
Wei-Yu Chi, Louise Ferrall, Chien-Fu Hung & T.-C. Wu
Johns Hopkins School of Medicine, 1550 Orleans St, CRB II – Room 309, Baltimore, MD, 21287, USA
You can also search for this author in PubMed Google Scholar
Contributions
YDL and WYC contributed to the conception of the manuscript and to the original draft preparation. JHS, LF, and CFH contributed to substantial review and preparation of the manuscript. TCW supervised the review. All authors read and approved the final manuscript.
Corresponding author
Correspondence to T.-C. Wu .
Ethics declarations
Ethics approval and consent to participate, consent for publication, competing interests.
Dr T.C. Wu is a co-founder of and has an equity ownership interest in Papivax LLC. Additionally Dr. Wu owns Papivax Biotech Inc. stock options and is a member of Papivax Biotech Inc.’s Scientific Advisory Board. This arrangement has been reviewed and approved by the Johns Hopkins University in accordance with its conflict of interest policies.
Additional information
Publisher's note.
Springer Nature remains neutral with regard to jurisdictional claims in published maps and institutional affiliations.
Rights and permissions
Open Access This article is licensed under a Creative Commons Attribution 4.0 International License, which permits use, sharing, adaptation, distribution and reproduction in any medium or format, as long as you give appropriate credit to the original author(s) and the source, provide a link to the Creative Commons licence, and indicate if changes were made. The images or other third party material in this article are included in the article's Creative Commons licence, unless indicated otherwise in a credit line to the material. If material is not included in the article's Creative Commons licence and your intended use is not permitted by statutory regulation or exceeds the permitted use, you will need to obtain permission directly from the copyright holder. To view a copy of this licence, visit http://creativecommons.org/licenses/by/4.0/ . The Creative Commons Public Domain Dedication waiver ( http://creativecommons.org/publicdomain/zero/1.0/ ) applies to the data made available in this article, unless otherwise stated in a credit line to the data.
Reprints and permissions
About this article
Cite this article.
Li, YD., Chi, WY., Su, JH. et al. Coronavirus vaccine development: from SARS and MERS to COVID-19. J Biomed Sci 27 , 104 (2020). https://doi.org/10.1186/s12929-020-00695-2
Download citation
Received : 30 October 2020
Accepted : 20 November 2020
Published : 20 December 2020
DOI : https://doi.org/10.1186/s12929-020-00695-2
Share this article
Anyone you share the following link with will be able to read this content:
Sorry, a shareable link is not currently available for this article.
Provided by the Springer Nature SharedIt content-sharing initiative
- Coronaviruses
- Vaccine development
Journal of Biomedical Science
ISSN: 1423-0127
- Submission enquiries: Access here and click Contact Us
- General enquiries: [email protected]
An Overview Of Vaccine Development, Approval, And Regulation, With Implications For COVID-19
Affiliations.
- 1 Aaron S. Kesselheim ([email protected]) is a professor of medicine and the director of the Program on Regulation, Therapeutics, and Law in the Division of Pharmacoepidemiology and Pharmacoeconomics, Department of Medicine, Brigham and Women's Hospital and Harvard Medical School, in Boston, Massachusetts.
- 2 Jonathan J. Darrow is an assistant professor of medicine in the Division of Pharmacoepidemiology and Pharmacoeconomics, Department of Medicine, Brigham and Women's Hospital and Harvard Medical School.
- 3 Martin Kulldorff is a professor of medicine in the Division of Pharmacoepidemiology and Pharmacoeconomics, Department of Medicine, Brigham and Women's Hospital and Harvard Medical School.
- 4 Beatrice L. Brown is a research assistant in the Division of Pharmacoepidemiology and Pharmacoeconomics, Department of Medicine, Brigham and Women's Hospital and Harvard Medical School.
- 5 Mayookha Mitra-Majumdar is a research scientist in the Division of Pharmacoepidemiology and Pharmacoeconomics, Department of Medicine, Brigham and Women's Hospital and Harvard Medical School.
- 6 ChangWon C. Lee is a research assistant in the Division of Pharmacoepidemiology and Pharmacoeconomics, Department of Medicine, Brigham and Women's Hospital and Harvard Medical School.
- 7 Osman Moneer is a medical student at the Yale School of Medicine, in New Haven, Connecticut.
- 8 Jerry Avorn is a professor of medicine in the Division of Pharmacoepidemiology and Pharmacoeconomics, Department of Medicine, Brigham and Women's Hospital and Harvard Medical School.
- PMID: 33211535
- DOI: 10.1377/hlthaff.2020.01620
The Food and Drug Administration (FDA) approves vaccines when their benefits outweigh the risks for their intended use. In this article we review the standard FDA approach to vaccine evaluation, which underpins its current approaches to assessment of vaccines to prevent coronavirus disease 2019 (COVID-19). The FDA has established pathways to accelerate vaccine availability before approval, such as Emergency Use Authorization, and to channel resources to high-priority products and allow more flexibility in the evidence required for approval, including accelerated approval based on surrogate markers of effectiveness. Among the thirty-five new vaccines approved in the US from 2006 through October 2020, about two-thirds of their pivotal trials used the surrogate outcome of immune system response, and only one-third evaluated actual disease incidence. Postapproval safety surveillance of new vaccines, and particularly vaccines receiving expedited approval, is crucial. This has generally been accomplished through such mechanisms as the Centers for Disease Control and Prevention (CDC) and FDA Vaccine Adverse Event Reporting System, the CDC Vaccine Safety Datalink, and the CDC Clinical Immunization Safety Assessment Project. Adverse events detected in this way may lead to changes in a vaccine's recommended use or withdrawal from the market. Regulatory oversight of new vaccines must balance speed with rigor to effectively address the pandemic.
- COVID-19 / prevention & control*
- COVID-19 Vaccines / pharmacology*
- Centers for Disease Control and Prevention, U.S. / standards
- Drug Approval / organization & administration*
- Immunization / adverse effects
- Patient Safety
- Pharmaceutical Preparations / standards*
- Risk Assessment
- United States
- United States Food and Drug Administration / standards*
- COVID-19 Vaccines
- Pharmaceutical Preparations
Quantifying the Social Value of a Universal COVID-19 Vaccine and Incentivizing Its Development
A booster of the COVID-19 vaccine targeting the prevailing Omicron variant did not become available in the United States until a year after the variant was first detected. This pattern of developing, testing, and distributing a variant-specific booster may become the default response to further waves of COVID-19 caused by new variants. An innovation with realistic scientific potential—a universal COVID-19 vaccine, effective against existing and future variants—could provide much more value by preempting new variants. Averaged across Monte Carlo simulations, we estimate the incremental value to the U.S. population of a universal COVID-19 vaccine to be $1.5–$2.6 trillion greater than variant-specific boosters (depending on how the arrival rate of variants is modeled). This social value eclipses the cost of an advance market commitment to incentivize the universal vaccine by several orders of magnitude.
The authors are grateful for helpful discussions with Willy Chertman, Jake Eberts, Josh Morrison, Stanley Plotkin, Alec Stapp, Nikki Teran, and Witold Więcek. This is a substantially revised and expanded version of our working paper: T. Kelly, R. Glennerster, C.M. Snyder (2023) “An advance market commitment to incentivize a universal coronavirus vaccine before the next variant,” Washington, DC: 1Day Sooner. This working paper, in turn, built on initial work by Eric Mannes, to whom we are especially indebted. Snyder is grateful to the Institute for Progress for funding. The views expressed herein are those of the authors and do not necessarily reflect the views of the National Bureau of Economic Research.
MARC RIS BibTeΧ
Download Citation Data
Working Groups
More from nber.
In addition to working papers , the NBER disseminates affiliates’ latest findings through a range of free periodicals — the NBER Reporter , the NBER Digest , the Bulletin on Retirement and Disability , the Bulletin on Health , and the Bulletin on Entrepreneurship — as well as online conference reports , video lectures , and interviews .
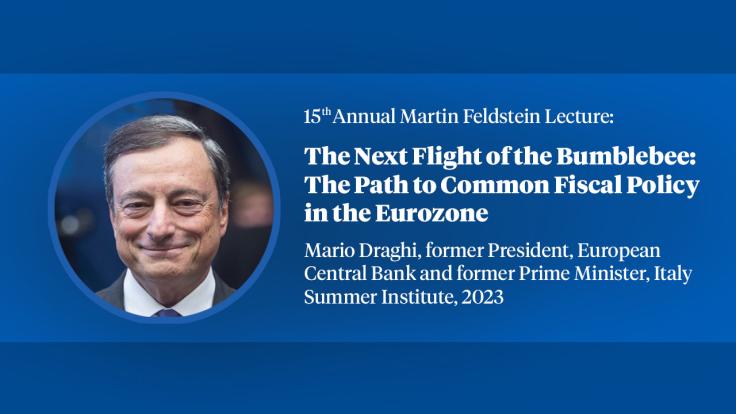
Economic Evaluation of COVID-19 Immunization Strategies: A Systematic Review and Narrative Synthesis
- Systematic Review
- Published: 10 April 2024
Cite this article
- Enxue Chang 1 na1 ,
- Haofei Li 1 na1 ,
- Wanji Zheng 1 na1 ,
- Lan Zhou 1 ,
- Yanni Jia 1 ,
- Yiyin Cao 1 ,
- Xiaoying Zhu 2 , 3 ,
- Juan Xu 4 ,
- Mao You 6 ,
- Kejun Liu 6 ,
- Mingsi Wang 1 &
- Weidong Huang ORCID: orcid.org/0009-0008-9580-6862 1
60 Accesses
Explore all metrics
This study aimed to systematically assess global economic evaluation studies on COVID-19 vaccination, offer valuable insights for future economic evaluations, and assist policymakers in making evidence-based decisions regarding the implementation of COVID-19 vaccination.
Searches were performed from January 2020 to September 2023 across seven English databases (PubMed, Web of Science, MEDLINE, EBSCO, KCL-Korean Journal Dataset, SciELO Citation Index, and Derwent Innovations Index) and three Chinese databases (Wanfang Data, China Science and Technology Journal, and CNKI). Rigorous inclusion and exclusion criteria were applied. Data were extracted from eligible studies using a standardized data collection form, with the reporting quality of these studies assessed using the Consolidated Health Economic Evaluation Reporting Standards 2022 (CHEERS 2022).
Of the 40 studies included in the final review, the overall reporting quality was good, evidenced by a mean score of 22.6 (ranging from 10.5 to 28). Given the significant heterogeneity in fundamental aspects among the studies reviewed, a narrative synthesis was conducted. Most of these studies adopted a health system or societal perspective. They predominantly utilized a composite model, merging dynamic and static methods, within short to medium-term time horizons to simulate various vaccination strategies. The research strategies varied among studies, investigating different doses, dosages, brands, mechanisms, efficacies, vaccination coverage rates, deployment speeds, and priority target groups. Three pivotal parameters notably influenced the evaluation results: the vaccine's effectiveness, its cost, and the basic reproductive number ( R 0 ). Despite variations in model structures, baseline parameters, and assumptions utilized, all studies identified a general trend that COVID-19 vaccination is cost-effective compared to no vaccination or intervention.
Conclusions
The current review confirmed that COVID-19 vaccination is a cost-effective alternative in preventing and controlling COVID-19. In addition, it highlights the profound impact of variables such as dose size, target population, vaccine efficacy, speed of vaccination, and diversity of vaccine brands and mechanisms on cost effectiveness, and also proposes practical and effective strategies for improving COVID-19 vaccination campaigns from the perspective of economic evaluation.
This is a preview of subscription content, log in via an institution to check access.
Access this article
Price includes VAT (Russian Federation)
Instant access to the full article PDF.
Rent this article via DeepDyve
Institutional subscriptions
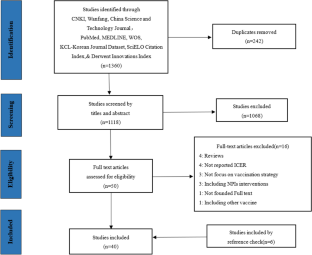
Vaezi A, Meysamie A. COVID-19 vaccines cost-effectiveness analysis: a scenario for Iran. Vaccines. 2021;10(1):37.
Article PubMed PubMed Central Google Scholar
WHO. WHO Director-General’s opening remarks at the media briefing on COVID-19–11 March 2020. https://www.who.int/director-general/speeches/detail/who-director-general-s-opening-remarks-at-the-media-briefing-oncovid-19---11-march-2020 .
WHO. Coronavirus disease (COVID-19) Weekly Epidemiological Updates and Monthly Operational Updates; 2023. https://www.who.int/emergencies/diseases/novel-coronavirus-2019/situation-reports .
Vysochyna A, et al. Impact of coronavirus disease COVID-19 on the relationship between healthcare expenditures and sustainable economic growth. Int J Environ Res Public Health. 2023;20(4):3049.
Di Fusco M, et al. Health outcomes and economic burden of hospitalized COVID-19 patients in the United States. J Med Econ. 2021;24(1):308–17.
Article PubMed Google Scholar
Keogh-Brown MR, et al. The impact of Covid-19, associated behaviours and policies on the UK economy: a computable general equilibrium model. SSM Popul Health. 2020;12: 100651.
John D, et al. Estimation of the economic burden of COVID-19 using disability-adjusted life years (DALYs) and productivity losses in Kerala, India: a model-based analysis. BMJ Open. 2021;11(8): e049619.
An X, et al. Economic burden of public health care and hospitalisation associated with COVID-19 in China. Public Health. 2022;203:65–74.
Article CAS PubMed Google Scholar
Jin H, et al. Economic burden of COVID-19, China, January-March, 2020: a cost-of-illness study. Bull World Health Organ. 2021;99(2):112–24.
Harrison EA, Wu JW. Vaccine confidence in the time of COVID-19. Eur J Epidemiol. 2020;35(4):325–30.
Article CAS PubMed PubMed Central Google Scholar
ANVISA. Bases técnicas para decisão do uso emergencial, Em caráter experimental de vacinas contra a COVID-19 .
Morales-Zamora G, et al. Cost-effectiveness analysis of strategies of COVID-19 vaccination in Colombia: comparison of high-risk prioritization and no prioritization strategies with the absence of a vaccination plan. Value Health Reg Issues. 2022;05(31):101–10.
Article Google Scholar
WHO. COVID-19 vaccine tracker and landscape; 2023. https://www.who.int/publications/m/item/draft-landscape-of-covid-19-candidate-vaccines .
Group, S. Shiyao Group's COVID-19 mRNA vaccine is included in emergency use in China; 2023. http://www.e-cspc.com/details/details_92_4894.html .
Kirwin E, et al. A net benefit approach for the optimal allocation of a COVID-19 vaccine. Pharmacoeconomics. 2021;39(9):1059–73.
World Health Organization. Adding a vaccine to a national immunization programme: decision and implementation. Geneva: World Health Organization; 2005.
Google Scholar
Walker DG, Hutubessy R, Beutels P. WHO Guide for standardisation of economic evaluations of immunization programmes. Vaccine. 2010;28(11):2356–9.
Utami AM, et al. Cost-effectiveness analysis of COVID-19 vaccination in low- and middle-income countries. J Multidiscip Healthc. 2022;15:2067–76.
Zhao JY, et al. Progress in research of economic evaluation of COVID-19 vaccination strategies. Zhonghua Liu Xing Bing Xue Za Zhi. 2022;43(4):460–5.
CAS PubMed Google Scholar
Utami AM, et al. Economic evaluation of COVID-19 vaccination: a systematic review. J Glob Health. 2023;13:06001.
Santoli G, et al. Incremental net benefit and incremental cost-effectiveness ratio of COVID-19 vaccination campaigns: systematic review of cost-effectiveness evidence. Vaccines (Basel). 2023;11(2):347.
Fu Y, et al. Cost-effectiveness of COVID-19 vaccination: a systematic review. J Evid Based Med. 2023;16(2):152–65.
Aidalina M, Khalsom S. COVID-19 vaccination: a systematic review of vaccination strategies based on economic evaluation studies. Med J Malaysia. 2023;78:411–20.
Page MJ, et al. The PRISMA 2020 statement: an updated guideline for reporting systematic reviews. BMJ. 2021;372: n71.
Moher D, et al. Preferred reporting items for systematic reviews and meta-analyses: the PRISMA statement. PLoS Med. 2009;6(7): e1000097.
International Monetary Fund. Consumer Price Index (CPI). https://data.imf.org/?sk=4ffb52b2-3653-409a-b471-d47b46d904b5&sid=1485878855236 .
International Monetary Fund. Representative exchange rates for selected currencies for December 2023. https://www.imf.org/external/np/fin/data/rms_mth.aspx?SelectDate=2023-12-31&reportType=REP .
Husereau D, et al. Consolidated health economic evaluation reporting standards 2022 (CHEERS 2022) statement: updated reporting guidance for health economic evaluations. Int J Technol Assess Health Care. 2022;38(1): e13.
Husereau D, et al. Consolidated Health Economic Evaluation Reporting Standards 2022 (CHEERS 2022) statement: updated reporting guidance for health economic evaluations. BMJ. 2022;376: e067975.
Husereau D, et al. Consolidated Health Economic Evaluation Reporting Standards (CHEERS) 2022 Explanation and Elaboration: a report of the ISPOR CHEERS II Good Practices Task Force. Value Health. 2022;25(1):10–31.
Du Y, et al. Economic evaluations of 13-valent pneumococcal conjugate vaccine: a systematic review. Expert Rev Vaccines. 2023;22(1):193–206.
Popay J, Roberts H, Sowden A, et al. Guidance on the conduct of narrative synthesis in systematic reviews. 1. A product from the ESRC methods programme version. 2006:b92.
Li R, et al. Cost-effectiveness analysis of BNT162b2 COVID-19 booster vaccination in the United States. Int J Infect Dis. 2022;03(119):87–94.
Kohli M, et al. The potential public health and economic value of a hypothetical COVID-19 vaccine in the United States: use of cost-effectiveness modeling to inform vaccination prioritization. Vaccine. 2021;39(7):1157–64.
Di Fusco M, et al. Public health impact of the Pfizer-BioNTech COVID-19 vaccine (BNT162b2) in the first year of rollout in the United States. J Med Econ. 2022;25(1):605–17.
Bartsch SM, et al. Lives and costs saved by expanding and expediting coronavirus disease 2019 vaccination. J Infect Dis. 2021;224(6):938–48.
Padula WV, et al. Economic value of vaccines to address the COVID-19 pandemic: a U.S. cost-effectiveness and budget impact analysis. J Med Econ. 2021;24(1):1060–9.
Shaker M, Abrams EM, Greenhawt M. A cost-effectiveness evaluation of hospitalizations, fatalities, and economic outcomes associated with universal versus anaphylaxis risk-stratified COVID-19 vaccination strategies. J Allergy Clin Immunol Pract. 2021;9(7):2658-2668.e3.
Fu Y, et al. Effectiveness and cost-effectiveness of inactivated vaccine to address COVID-19 pandemic in China: evidence from randomized control trials and real-world studies. Front Public Health. 2022;07(10): 917732.
Xiong X, et al. Economic value of vaccines to address the COVID-19 pandemic in Hong Kong: a cost-effectiveness analysis. Vaccines (Basel). 2022;10(4):495.
Wang WC, et al. Economic evaluation for mass vaccination against COVID-19. J Formos Med Assoc. 2021;06(120):S95–105.
Zhou H, et al. Cost-effectiveness analysis of vaccination against COVID-19 in China. Front Public Health. 2023;11: 1037556.
Du M, et al. Cost-effectiveness analysis of COVID-19 inactivated vaccines in reducing the economic burden of ischaemic stroke after SARS-CoV-2 infection. Vaccines. 2023;11(5):957.
Fu Y, et al. Cost-effectiveness of COVID-19 sequential vaccination strategies in inactivated vaccinated individuals in China. Vaccines (Basel). 2022;10(10):1712.
Wang Y, et al. Assessing the cost-effectiveness of COVID-19 vaccines in a low incidence and low mortality setting: the case of Thailand at start of the pandemic. Eur J Health Econ. 2022;08:1–14.
Sirison K, et al. Cost-effectiveness analysis of COVID-19 vaccine booster dose in the Thai setting during the period of omicron variant predominance. Trop Med Infect Dis. 2023;8(2):91.
Suphanchaimat R, et al. Prioritization of the target population for coronavirus disease 2019 (COVID-19) vaccination program in Thailand. Int J Environ Res Public Health. 2021;18(20): 10803.
Du Z, et al. Cost effectiveness of fractional doses of COVID-19 vaccine boosters in India. Medicine (N Y). 2023;4(3):182-190.e3.
Du ZW, et al. Modeling comparative cost-effectiveness of SARS-CoV-2 vaccine dose fractionation in India. Nat Med. 2022;28(5):934.
Marco-Franco JE, et al. Simplified mathematical modelling of uncertainty: cost-effectiveness of COVID-19 vaccines in Spain. Mathematics. 2021;9(5):566.
Lopez F, et al. A cost-benefit analysis of COVID-19 vaccination in Catalonia. Vaccines. 2021;10(1):59.
Siedner MJ, et al. Cost-effectiveness of COVID-19 vaccination in low- and middle-income countries. J Infect Dis. 2022;226:1887–96.
Savinkina A, et al. Estimating deaths averted and cost per life saved by scaling up mRNA COVID-19 vaccination in low-income and lower-middle-income countries in the COVID-19 Omicron variant era: a modelling study. BMJ Open. 2022;12(9): e061752.
Jiang Y, Cai D, Shi S. Economic evaluations of inactivated COVID-19 vaccines in six Western Pacific and South East Asian countries and regions: a modeling study. Infect Dis Model. 2021;7(1):109–21.
PubMed PubMed Central Google Scholar
Liu Y, et al. Assessing the impacts of COVID-19 vaccination programme’s timing and speed on health benefits, cost-effectiveness, and relative affordability in 27 African countries. BMC Med. 2023;21(1):85.
Reddy KP, et al. Clinical outcomes and cost-effectiveness of COVID-19 vaccination in South Africa. Nat Commun. 2021;12(1):6238.
Orlewska K, Wierzba W, Sliwczynski A. Cost-effectiveness analysis of COVID-19 vaccination in Poland. Arch Med Sci. 2021;18(4):1021–30.
Edejer TT-T, Baltussen RM, Adam T, Hutubessy R, Acharya A, Evans D, et al. Making choices in health: WHO guide to cost-effective analysis. Geneva: WHO; 2003.
Debrabant K, Grønbæk L, Kronborg C. The cost-effectiveness of a COVID-19 vaccine in a Danish context. Clin Drug Investig. 2021;41(11):975–88.
Mungmunpuntipantip R, Wiwanitkit V. Expected cost effectiveness of the fourth dose of COVID-19 vaccine against the omicron variant of COVID-19: a preliminary report. Int J Physiol Pathophysiol Pharmacol. 2022;14(4):272–5.
CAS PubMed PubMed Central Google Scholar
Fernandes RRA, et al. Cost utility of vaccination against COVID-19 in Brazil. Value Health Reg Issues. 2022;01(31):18–24.
Yaroslavovych TB, et al. Pharmaco economics analysis of COVID-19 vaccines in Ukraine. J Pharm Res Int. 2021;06:140–7.
Orangi S, et al. Epidemiological impact and cost-effectiveness analysis of COVID-19 vaccination in Kenya. BMJ Glob Health. 2022;7(8): e009430.
Sandmann FG, et al. The potential health and economic value of SARS-CoV-2 vaccination alongside physical distancing in the UK: a transmission model-based future scenario analysis and economic evaluation. Lancet Infect Dis. 2021;21(7):962–74.
MacIntyre CR. Navigating post-vaccine COVID-19 futures in the health and economic context. Lancet Infect Dis. 2021;21(7):893–4.
Mahmud I, et al. The health belief model predicts intention to receive the COVID-19 vaccine in Saudi Arabia: results from a cross-sectional survey. Vaccines (Basel). 2021;9(8):864.
Rodrigues CMC, Plotkin SA. Impact of vaccines; health, economic and social perspectives. Front Microbiol. 2020;11:1526.
Yue MA, Ke XT, Liang XY, et al. Application of transmission dynamics model in economic evaluation on HPV vaccine vaccination: a brief introduction. Chin J Public Health. 2021;37(12):1742–5.
Ciotti M, et al. The COVID-19 pandemic: viral variants and vaccine efficacy. Crit Rev Clin Lab Sci. 2021;59(1):66–75.
Mateus J, et al. Selective and cross-reactive SARS-CoV-2 T cell epitopes in unexposed humans. Science. 2020;370(6512):89–94.
Mascola JR, Graham BS, Fauci AS. SARS-CoV-2 viral variants-tackling a moving target. JAMA. 2021;325(13):1261–2.
Tregoning JS, et al. Progress of the COVID-19 vaccine effort: viruses, vaccines and variants versus efficacy, effectiveness and escape. Nat Rev Immunol. 2021;21(10):626–36.
Haddad FS, McLawhorn AS. Guidelines for reporting health economics research. Bone Joint J. 2016;98:147–51.
World Health Organization. WHO guide for standardisation of economic evaluations of immunization programmes. Paris: World Health Organization; 2019.
Bertram MY, et al. Methods for the economic evaluation of health care interventions for priority setting in the health system: an update from WHO CHOICE. Int J Health Policy Manag. 2021;10(11):673–7.
Drummond M, Sculpher M, Claxton K, Stoddart GL, Torrance GW. Methods for the economic evaluation of health care programmes. Oxford: Oxford University Press; 2015. p. 42–4.
Shields GE, Elvidge J. Challenges in synthesising cost-effectiveness estimates. Syst Rev. 2020;9(1):289.
Download references
Author information
Enxue Chang, Haofei Li and Wanji Zheng have contributed equally to this work and share first authorship.
Authors and Affiliations
School of Health Management, Harbin Medical University, Harbin, China
Enxue Chang, Haofei Li, Wanji Zheng, Lan Zhou, Yanni Jia, Wen Gu, Yiyin Cao, Mingsi Wang & Weidong Huang
School of Elderly Care Services and Management, Nanjing University of Chinese Medicine, Nanjing, Jiangsu, China
Xiaoying Zhu
Nossal Institute for Global Health, School of Population and Global Health, The University of Melbourne, Melbourne, VIC, Australia
Cancer Hospital Chinese Academy of Medical Sciences, Shenzhen Center, Shenzhen, China
Shenzhen Health Capacity Building and Continuing Education Center, Shenzhen, China
National Health Development Research Center, Beijing, 100191, China
Mao You & Kejun Liu
You can also search for this author in PubMed Google Scholar
Corresponding authors
Correspondence to Xiaoying Zhu , Kejun Liu , Mingsi Wang or Weidong Huang .
Ethics declarations
This research was funded by the Beijing Natural Science Foundation-Haidian Original Innovation Joint Fund (L212012).
Conflict of Interest
The authors declare that they have no known competing financial interests or personal relationships that could have appeared to influence the work reported in this paper.
Data Availability
Not applicable.
Ethics Approval
Consent to participate, consent for publication, code availability, author contributions.
Enxue Chang, Weidong Huang, Mao You, Kejun Liu, and Mingsi Wang contributed to the study conception and design. Enxue Chang, Haofei Li, and Wanji Zheng performed screening, full text selection, and data extraction. Enxue Chang, Haofei Li, Wanji Zheng, Lan Zhou, and Yanni Jia conducted the quality appraisal of the studies. Mingsi Wang, Wen Gu, Yiyin Cao, Juan Xu, and Bo Liu contributed to the data interpretation. The first draft of the manuscript was written by Enxue Chang, Weidong Huang, and Xiaoying Zhu, and all authors contributed to the critical revision of the manuscript for intellectual content and approved the final draft submitted for publication.
Additional information
Weidong Huang is the leading corresponding author.
Supplementary Information
Below is the link to the electronic supplementary material.
Supplementary file1 (DOCX 209 KB)
Rights and permissions.
Springer Nature or its licensor (e.g. a society or other partner) holds exclusive rights to this article under a publishing agreement with the author(s) or other rightsholder(s); author self-archiving of the accepted manuscript version of this article is solely governed by the terms of such publishing agreement and applicable law.
Reprints and permissions
About this article
Chang, E., Li, H., Zheng, W. et al. Economic Evaluation of COVID-19 Immunization Strategies: A Systematic Review and Narrative Synthesis. Appl Health Econ Health Policy (2024). https://doi.org/10.1007/s40258-024-00880-6
Download citation
Accepted : 17 March 2024
Published : 10 April 2024
DOI : https://doi.org/10.1007/s40258-024-00880-6
Share this article
Anyone you share the following link with will be able to read this content:
Sorry, a shareable link is not currently available for this article.
Provided by the Springer Nature SharedIt content-sharing initiative
- Find a journal
- Publish with us
- Track your research
SYSTEMATIC REVIEW article
How has research on the effectiveness and safety of covid-19 vaccination been evaluated: a scope review with emphasis on coronavac.
- 1 Grupo de Epidemiología, Universidad de Antioquia, Medellín, Colombia
- 2 Grupo de Enfermedades Tropicales y Resistencia Bacteriana, Universidad del Sinú, Montería, Colombia
- 3 Grupo de investigación EMAP - Estadística y Matemáticas Aplicadas, Pontificia Universidad Javeriana, Cali, Colombia
- 4 Grupo de Investigación, Secretaría de Salud Distrital, Cali, Colombia
- 5 Grupo de Investigación Clínica - PECET (GIC-PECET), Universidad de Antioquia, Medellín, Colombia
- 6 Grupo de Investigación en Economía, Gestión y Salud, ECGESA. Pontificia Universidad Javeriana, Cali, Colombia
- 7 Departamento de Salud pública y Epidemiología, Pontificia Universidad Javeriana, Cali, Colombia
Introduction: The control of the COVID-19 epidemic has been focused on the development of vaccines against SARS-CoV-2. All developed vaccines have reported safety and efficacy results in preventing infection and its consequences, although the quality of evidence varies depending on the vaccine considered. Different methodological designs have been used for their evaluation, which can influence our understanding of the effects of these interventions. CoronaVac is an inactivated vaccine, and it has been assessed in various studies, including clinical trials and observational studies. Given these differences, our objective was to explore the published information to answer the question: how has the efficacy/effectiveness and safety of CoronaVac been evaluated in different studies? This is to identify potential gaps and challenges to be addressed in understanding its effect.
Methods: A scoping review was carried out following the methodology proposed by the Joanna Briggs Institute, which included studies carried out in humans as of 2020, corresponding to systematic reviews, clinical trials, analytical or descriptive observational studies, in which the effectiveness and/or safety of vaccines for COVID19 were evaluated or described. There were no age restrictions for the study participants.
Results: The efficacy/effectiveness and safety of this vaccine was assessed through 113 studies. Nineteen corresponded to experimental studies, 7 of Phase II, 5 of Phase IV, and 4 were clinical trials with random assignment. Although some clinical trials with random assignment have been carried out, these have limitations in terms of feasibility, follow-up times, and with this, the possibility of evaluating safety outcomes that occur with low frequencies. Not all studies have used homogeneous methods of analysis. Both the prevention of infection, and the prevention of outcomes such as hospitalization or death, have been valued through similar outcomes, but some through multivariate analysis of dependencies, and others through analysis that try to infer causally through different control methods of confounding.
Conclusion: Published information on the evaluation of the efficacy/effectiveness and safety of the CoronaVac is abundant. However, there are differences in terms of vaccine application schedules, population definition, outcomes evaluated, follow-up times, and safety assessment, as well as non-standardization in the reporting of results, which may hinder the generalizability of the findings. It is important to generate meetings and consensus strategies for the methods and reporting of this type of studies, which will allow to reduce the heterogeneity in their presentation and a better understanding of the effect of these vaccines.
1 Introduction
Starting from the first reports coming from China and from countries in Europe and Asia, about the infection produced by SARS-CoV-2, its high contagion, and lethality of up to 14% in older adults, and the subsequent declaration of a COVID-19 pandemic, and together with the measures established by the healthcare authorities to manage the disease, efforts began to develop effective and safe vaccines that would contribute to speeding up the control of this health condition, through the reduction of infections, complications, and deaths associated with this disease ( 1 ).
For this reason, pandemic control efforts have focused on developing vaccines against SARS-CoV-2 that are capable of acting against infection, disease, or transmission, and thus contribute to disease control ( 2 ). In this context, different research groups have developed vaccines using different platforms, including mRNA, viral vectors, and inactivated viruses ( 3 ).
Unlike most drugs, whose benefits are limited to the individual taking them, vaccines have the potential to produce far-reaching effects on general public health and well-being, cognitive development, and, ultimately, economic productivity ( 4 ). However, the global advances in vaccination coverage achieved during the first years of the 21st century have been threatened by the emergence of anti-vaccination groups that have questioned vaccine efficacy to create public distrust of vaccines and immunization programs. This requires an adequate and conscious evaluation of both the efficacy/effectiveness and the different aspects that can affect the safety of the people who receive them ( 5 ).
In general, vaccines that have gained approval for human use have been effective in preventing COVID-19, particularly in preventing severe disease and death. However, reports on their implementation are mainly based on follow-up studies of the adult population ( 6 ). Additionally, if the vaccination prevents symptoms from developing and asymptomatic infections are less likely to be discovered than symptomatic ones, it is feasible that the effectiveness against any infection has been overstated. A competitive tendency toward underestimate arises when estimates are based on tests with inadequate specificity, particularly when testing are conducted more frequently than has been estimated for various COVID-19 vaccinations ( 7 ).
All vaccines seem to be safe and efficacious against all variations of interest in preventing hospitalization, death, and severe COVID-19; however, the quality of the data differs significantly between the vaccines under consideration ( 8 ).
Different methodological designs have been used to evaluate the effectiveness and safety of vaccines for COVID-19. Most clinical trials were carried out before the appearance of variants of concern, and the duration, subgroups evaluated, and analysis methods were not homogeneous between vaccines, creating uncertainty about some effects and comparisons ( 9 ).
CoronaVac is an inactivated whole-virus vaccine against COVID-19 adjuvanted with aluminum hydroxide created from African green monkey kidney cells (Vero cells) inoculated with SARS-CoV-2 (strain CN02). The Chinese company Sinovac Biotech developed the vaccine, and on June 1, 2021, the World Health Organization (WHO) approved the vaccine for emergency use ( 10 ). Using two 3 μg doses of CoronaVac, the overall efficacy for avoiding symptomatic COVID-19 (before the emergence of concerning variations) has been assessed at 67.7% (95%CI: 35.9 to 83.7%) ( 10 ). Compared to COVID-19 prevention, its impact in preventing hospital stays, ICU admissions, and fatalities has been much stronger. Three-dose regimens have also been shown to raise seroconversion levels of neutralizing antibodies, even against variants like Omicron. Few serious vaccine-related adverse reactions have been reported ( 10 ).
However, given the differences that may exist in the methods used to assess the efficacy, effectiveness, and safety of vaccines against COVID-19, our objective was to explore the published research on COVID-19 vaccines, focusing on CoronaVac, in order to answer the question: How has the efficacy/effectiveness and safety of CoronaVac been assessed in different designs and study phases of the vaccines used to control COVID-19?
A scoping review was carried out under a protocol registered in the Open Science Framework (OSF; osf.io/aeut4), and following the methodology proposed by the Joanna Briggs Institute ( 11 ), which included studies carried out in humans as of 2020, corresponding to systematic reviews, clinical trials, and analytical or descriptive observational studies in which the effectiveness and/or safety of vaccines for COVID19 were evaluated or described. There were no age restrictions on the study participants.
Abstracts from congresses were not evaluated because they had not been subjected to systematic peer evaluation at the time, nor were studies published in languages other than English or Spanish.
2.1 Search methods for study identification
To identify potentially relevant articles for review, the following databases were searched, starting from 2020: MEDLINE, EMBASE, LILACS, Scopus, and Cochrane.
The following valid strategy was used for MEDLINE through PubMed and then adapted to other databases:
(((SARS-CoV-2[MeSH Terms]) OR (COVID-19[MeSH Terms])) OR (Coronavirus[MeSH Terms])) AND ((COVID-19 Vaccines[MeSH Terms]) OR (Coronavirus vaccines[Title/Abstract])).
The full search strategy is presented in the Supplementary material .
2.2 Study selection
The initial screening of the studies was independently performed by two reviewers in pairs (PA-AG and PR-SR). The RIS files of each database were uploaded to Rayyan software ( 12 ). Disagreements were resolved by a third author (JA).
Both reviewers assessed all titles and abstracts and excluded those considered irrelevant for the review, those not meeting the inclusion criteria, or because they were duplicates. Subsequently, 15 reviewers independently (JA, PA, DA, AC, AG, LL, LM, DO, GQ, SR, CR, PR, MS, CT, MA) evaluated the full text of the studies to verify the eligibility criteria. A cross-review was carried out for studies evaluating CoronaVac by four reviewers (PA, AG, PR, and SR).
2.3 Variable
Of the definitively selected studies, the following variables were extracted in a paired form: (i) type of study, (ii) population studied, (iii) intervention (vaccine) evaluated, (iv) control, (v) follow-up time, (vi) efficacy and/or effectiveness outcomes, and (vii) safety outcomes.
2.4 Data synthesis
For each outcome, a description of the results was made following the description in the document and/or Supplementary material of the article.
3.1 Study selection
The search identified 42,813 titles for the initial evaluation, of which 40,372 were excluded after a review of the title, abstract, and possible duplication. A total of 2,441 full texts were reviewed to verify the eligibility criteria, of which 1,685 were included in the synthesis ( Figure 1 ; Supplementary material ).
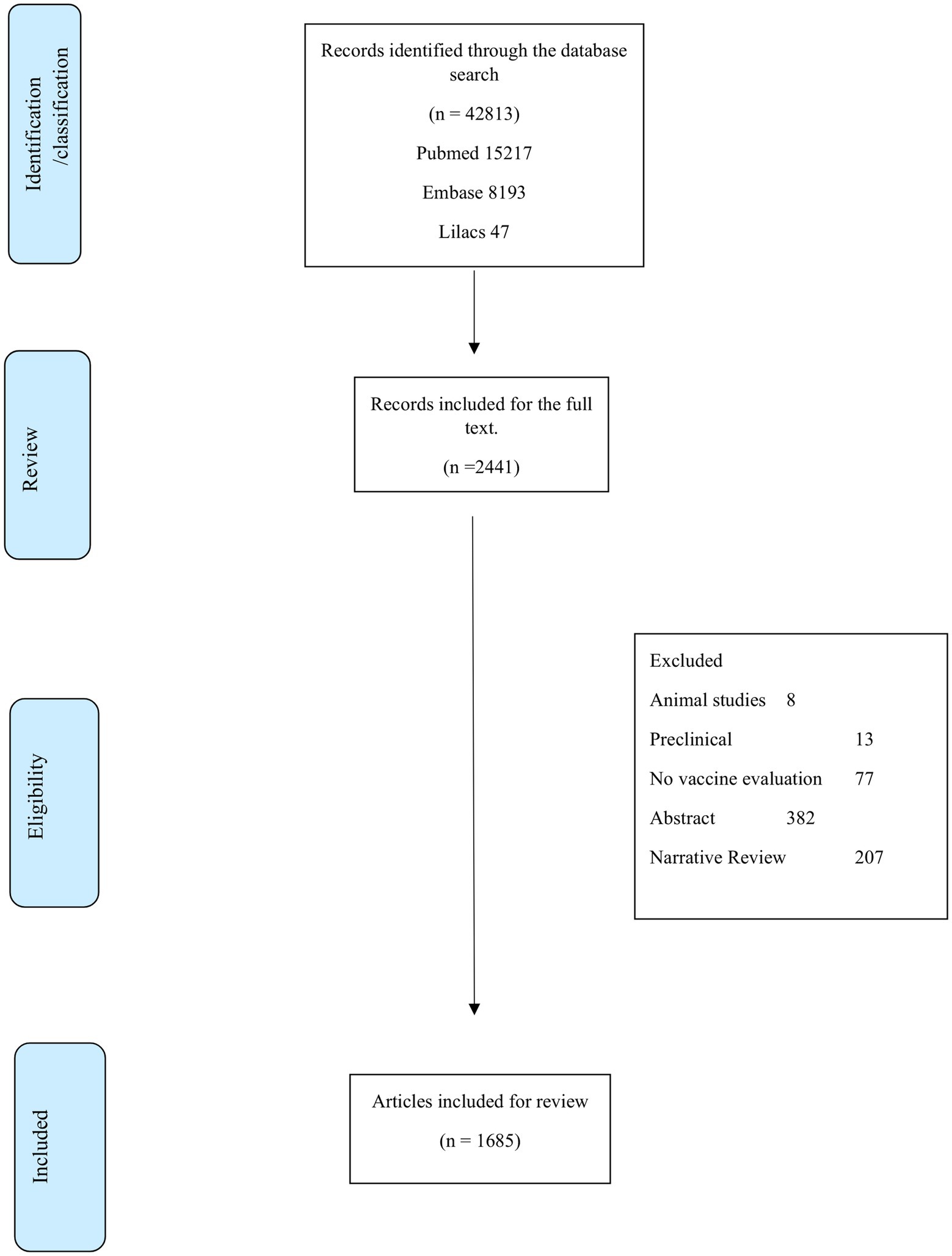
Figure 1 . Flow diagram of the literature review process.
3.2 Synthesis of the results
One hundred vaccines were evaluated through randomized clinical trials (RCT). The other studies corresponded to observational studies, 705 (43.9%) analytical studies, and mainly cohort studies (467; 29.1%). Three hundred and seventy-seven patients (23.5%) were series or case reports.
One hundred twenty-six studies (7.8%) did not specify the vaccine evaluated. Other studies have evaluated one or more specific vaccines. Seven hundred thirty-two studies did not include a vaccine or a control group. Two hundred and thirty-eight evaluated several types of vaccines, and 160 compared a vaccine against a placebo. The number of patients or vaccine doses evaluated in each study went from one (case report) to 306,473,169 doses of applied vaccines ( 13 ).
Regarding the population assessed, 44.4% of the studies evaluated the effects of vaccines on adults. 3.4% in adults and adolescents, 2% in adolescents, 1.2% in immunosuppressed individuals, 1.2% in children, 0.9% in pregnant women, and 0.25% in people living with HIV. The overall monitoring time ranged from hours to 6 months; this difference occurred between studies that evaluated immunological outcomes, which could occur within hours or days, and those that evaluated clinical outcomes.
A total of 15.1% of the studies evaluated the effectiveness or efficacy of vaccines by evaluating their effects on preventing infection, hospitalization, or death from infection. 59.1% of the studies corresponded to the description of safety events. The events were described heterogeneously. In some studies, they are only recorded as “mild adverse events” or “mild systemic events.” Few studies reported specific events such as myocarditis, and hepatic or allergic alterations. Of the studies, 25.8% described immunological outcomes, 368 studies through the measurement of antibodies, and 64 through the effects mediated by cellular immunity.
3.2.1 CoronaVac
The efficacy, effectiveness, and safety of this vaccine have been assessed in 113 studies. Nineteen corresponded to experimental studies, seven of Phase II, five of Phase IV, and four were clinical trials with random assignment, carried out in adults in Chile, Indonesia, and Turkey ( 14 – 17 ), comparing the effect of the vaccine versus placebo. The other studies were observational studies, most of which were case reports, case series, or descriptions of cohorts. Of these, 45.1% were conducted in Asia, 23% in Latin America, and 22.1% in Europe, mainly in Turkey (of 27/29 European studies).
As for the population, 87.6% of the studies were conducted in adults, while the representation of studies in pregnant women, children, immunosuppressed people, or people living with HIV ranged between 0.9 and 3.5% of the studies.
Sixty studies (53.1%) evaluated the effect of CoronaVac in a control group. The others were case reports or descriptions of cohorts without comparison. Of these, 42 (70%) described events in patients who received CoronaVac and another vaccine, without performing an effectiveness or efficacy analysis. Other studies evaluated the efficacy and effectiveness by measuring the effect of preventing hospitalization, death, or COVID. Of the total, 34 studies evaluated CoronaVac (30.1%) and described some immune outcomes.
Although the objective of the review was not to assess the effectiveness of the vaccine, but rather how it has been evaluated, the results of some of the identified studies are shown below in order to present relevant information about the methods used and possible differences between them, which lead to discussing the effect that this can have on the analysis and use of CoronaVac and other vaccines. More details on the results of the identified studies can be found in the Supplementary material section.
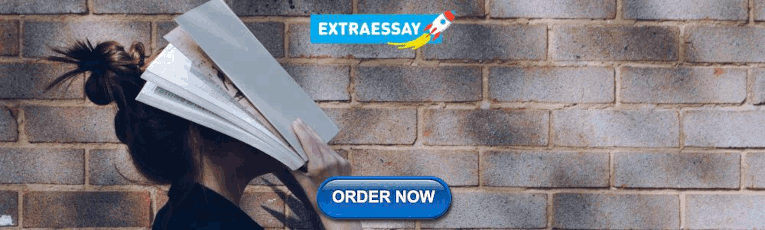
3.2.2 Efficacy/effectiveness of CoronaVac
3.2.2.1 prevention of covid-19.
Cheng et al. ( 18 ) evaluated the effectiveness of BNT162B2 and CoronaVac in patients with chronic kidney disease in Hong Kong. 28,374 people were not vaccinated, 27,129 received two doses of BNT162b2, and 47,640 received two doses of CoronaVac in this retrospective cohort analysis. Following inverse probability of treatment weighting with 1% extreme values, a cohort that was well-balanced and had a standardized mean difference of less than 0.1 was generated.
The effectiveness of CoronaVac on Turkish healthcare professionals was assessed by Can et al. ( 19 ). 4,067 medical personnel worked at a University Hospital in Istanbul, where this retrospective cohort study was carried out. In the fully vaccinated group, the follow-up period was defined as beginning 14 days following the second dose. If PCR test findings were positive or the trial came to an end, healthcare personnel were excluded. Healthcare personnel who were not vaccinated were prohibited from participating in any COVID-19 vaccination. The vaccine’s unadjusted and adjusted effectiveness were calculated using the incidence rate ratio and Cox regression. 29% of the healthcare staff had not received any vaccinations, whereas 71% had received all recommended doses.
Jara et al. ( 20 ) conducted an evaluation of a prospective, observational, national-level cohort of individuals (≥ 16 years) associated with the Fondo Nacional de Salud insurance program in Chile. They used individual-level data to assess the efficacy of booster vaccines, namely BNT162B2 (Pfizer-Biontech), AZD1222 (Oxford-AstraZeneca), and CoronaVac (Syovac Biotech), in individuals who had completed a primary immunization schedule with CoronaVac, in comparison to those who had not received any vaccinations. The hazard ratios were estimated using inverse probability-weighted and stratified survival regression models that took into account the time-varying vaccination status and adjusted for pertinent clinical, socioeconomic, and demographic confounders. An estimate was made of the change in risk associated with the primary immunization series and booster shot from being unvaccinated to vaccinated. 11,174,257 persons in total fulfilled the trial’s eligibility conditions; of these, 4,127,546 finished the two doses of the CoronaVac primary immunization regimen and got a booster dose during the study period. 2,019,260 (48.9%) individuals received a BNT162b2 booster, 186,946 (4.5%) received a homologous booster with CoronaVac, and 1,921,340 (46–5%) participants received an AZD1222 booster. The weighted stratified Cox model was utilized to compute the modified vaccination efficacy in preventing COVID-19.
Utilizing hospitalization, vaccination, and National COVID-19 notification data, Cerqueira-Silva et al. ( 21 ) conducted a case–control study in Brazil to evaluate the efficacy of four vaccines (CoronaVac [synovac], ChAdOx1 nCoV-19 [AstraZeneca], Ad26.COV2.S [Janssen], and BNT162b2 [Pfizer-Bionntech]) in individuals with laboratory-confirmed prior SARS-COV-2 infection. The probabilities of test positivity and the likelihood of hospitalization or death from COVID-19 were compared based on vaccination status and the amount of time that had passed from the first or second dose of vaccinations using multivariable conditional logistic regression.
The same authors conducted a similar study in Brazil ( 22 ), using linked national Brazilian databases to conduct a negative-test design study with nearly 14 million participants (~ 16 million tests) to estimate the effectiveness of the CoronaVac vaccine over time and the BNT162B2 booster vaccination against severe COVID-19 outcomes (hospitalization or death) and severe acute respiratory syndrome, as confirmed by RT-PCR (SARS-COV-2).
To evaluate the effectiveness of homologous and heterologous boosters against COVID-19 in the context of OMICRON, Ranzani et al. ( 23 ) conducted a nationwide case–control study (with negative PCR results) to assess homologous and heterologous (BNT162B2) booster doses in adults who received two doses of CoronaVac in Brazil in the OMICRON context.
A case–control research was carried out in Thailand by Sritipsukho et al. ( 24 ) to assess the efficacy of various vaccination regimens in preventing COVID-19 during the time when the delta variant was the predominant causing virus (≥ 95%). By correcting for individual demographic and clinical factors, the efficacy of vaccines was assessed.
3.2.2.2 Prevention of hospitalization and death
Cheng et al. ( 18 ) found that both vaccines reduced hospitalization and death related to COVID-19, which was the opposite of the outcome of preventing COVID-19 infection. The vaccination efficacy for BNT162b2 users was 64% (95% CI: 57–69%) for hospitalization associated to COVID-19 and 86% (95% CI: 80–90%) for COVID-19-related death. Regarding hospitalization and death associated to COVID-19, the vaccine efficacy for CoronaVac was 44% (95% CI: 37–49%) and 70% (95% CI: 64–75%), respectively.
In the Jara et al. ( 20 ) study, the adjusted effectiveness of the vaccine against hospitalization due to COVID-19, ICU admission, and death was 86.3% (83.7–88.5), 92.2% (88.7–94.6), and 86.7% (80.5–91.0) for a CoronaVac homolog booster; 96.1% (95.3–96.9), 96, 2% (94.6–97.3), and 96.8% (93.9–98.3) for a BNT162b2 booster; and 97.7% (97.3–98.0), 98.9% (98.5–99.2), and 98.1% (97.3–98.6) for an AZD1222 booster, respectively.
In Brazil ( 21 ), the effectiveness against hospitalization or death 14 or more days after the completion of the vaccination schedule was 81.3% (75.3–85.8) for CoronaVac, 89.9% (83.5–93.8) for ChAdOx1 nCoV-19, and 57.7% (−2.6–82.5) for Ad26.COV2.S, and 89.7% (54.3–97.7) for BNT162b2.
3.2.2.3 Immunological outcomes
Bueno et al. ( 14 ), conducting a randomized placebo-controlled clinical trial in Chile, assessed the effectiveness of CoronaVac by assigning participants to either a placebo or two doses of CoronaVac spaced 2 weeks apart. Enrollments totaled 434, with 397 individuals in the 18–59 age range and 37 in the 60+ age range. 81 subjects had hemoral assessments. 2 and 4 weeks after the second dosage, respectively, the seroconversion rates for specific anti-S1-receptor binding domain (RBD) immunoglobulin G (IgG) were 82.22 and 84.44% in the 18–59 years age group and 62, 69 and 70.37% in the ≥60 years age group. A notable rise in the amount of neutralizing antibodies in circulation was noted two and 4 weeks following the second dosage. 47 participants had their cells evaluated. After stimulation with Mega Pools of SARS-CoV-2 peptides, a notable increase in T cell responses was seen, as evidenced by the release of interferon-γ (IFN-γ).
According to Zeng et al. ( 25 ) the following were the findings of two single-center, double-blind, randomized, placebo-controlled phase II clinical trials: adults from Jiangsu, China, aged 18 to 59 years were first assigned (1:1) into two vaccination schedule cohorts: one for the days 0 and 14 of vaccination (cohort 1), and another for the days 0 and 28 of vaccination (cohort 2). Each cohort was then randomly assigned (2:2:1) to either a placebo group or a 3 μg or 6 μg dose of CoronaVac. A third dose was given to half of the participants in each cohort 6 months after the second dose, and an additional dose was given to the other half of the individuals 28 days following the second dose, as a result of a protocol revision. In a separate phase II experiment carried out in Hebei, China, individuals who met the eligibility criteria of 60 years or above were randomized to receive three injections of 1.5, 3, or 6 μg of vaccine or a placebo. The first two doses of the vaccine were given 28 days apart, while the second and third doses were given 6 months apart. For the per-protocol population (those who finished their allotted third dose), the primary research outcomes were geometric mean titers (GMTs), geometric mean increments (GMIs), and seropositivity of neutralizing antibodies to SARS-CoV-2. Out of the 600 participants, who were between the ages of 18 and59, 540 (90%) were qualified for a third dose. Of these, 269 (50%) received the third primary dose (cohorts 1a-14d-2 m and 2a-28d-2 m) 2 months after the second dose, and 271 (50%) received a booster dose 8 months later (cohorts 1b-14d-8 m and 2b-28d-8 m). For the 1b-14d-8 m cohort ( n = 53; GMT 3.9 [95% CI 3.1–5.0]) and 2b-28d-8 m cohort ( n = 49; GMT 6.8 [95% CI 5.2–8.8]), neutralizing antibody titers elicited by the first two treatments in the 3 μg group declined after 6 months to close or below the seropositive cut-off point (GMT of 8). The GMTs measured 14 days later increased to 137.9 (95% CI: 99.9–190.4) for the 1b-14d-8 m cohort and to 143.1 (110.8–184.7) 28 days later for the 2b-28d-8 m cohort when a booster dose was administered 8 months following a second dose. After the principal third dosage, GMTs increased somewhat in cohorts 1a-14d-2 m (n = 54) and 2a-28d-2 m ( n = 53). In cohort 1a, GMTs increased from 21.8 (95% CI: 17.3–27.6) on day 28 after the second dose to 45.8 (35.7–58.9) on day 28 after the third dose. Six months following the third dose, GMTs had dropped to almost the positive threshold: in the 1a-14d-2 m group, they were 9.2 (95% CI 7.1–12.0), while in the 2a-28d-2 m cohort, they were 10.0 (7.3–13.7). Similarly, 6 months following the initial two-dose series, neutralizing antibody titers dropped to almost or below the seropositive threshold among people 60 years of age or older who received booster doses (303 [87%] of 350 participants were eligible for a third dosage). Eight months following the second treatment, which markedly raised neutralizing antibody concentrations, a third dose was administered: After the second dose on day 28, GMTs climbed to 42.9 (95% CI: 31.0–59.4), and after the third dose on day 28 ( n = 29), GMTs increased to 158.5 (96.6–259.2).
Chantasrisawad et al. ( 26 ) assessed healthy children aged 5 to 11 who were given two intramuscular doses of either Covilo or CoronaVac and 10 μg of BNT162b2. Neutralizing antibodies against the Omicron version were assessed using a pseudovirus neutralization test (pVNT, ID50) and a surrogate viral neutralization test (sVNT, % inhibition) 14–21 days following the booster. The antibody responses were contrasted with those of a concurrent cohort of kids who got two BNT162b2 doses separated by 3 weeks. A total of 59 children, consisting of 20 CoronaVac recipients and 39 Covilo recipients, were registered between April and May 2022, with a mean age (SD) of 8.5 years (1.7). The primary series’ median interval was 49 days, with an interquartile range of 33–51. Following the booster, the geometric means (MG) of pVNT and sVNT were 499 (95%CI: 399–624) and 72.2% inhibition (95%CI: 67.2–77.6), respectively. From zero to 72 %, the percentage of kids with sVNT against Omicron strain ≥68% inhibition rose. In comparison to the parallel cohort, the geometric mean ratios (GMR) of sVNT and pVNT were 4.3 and 12.2, respectively. In comparison to children who received a booster dosage between 4 and 6 weeks, the GMR of sVNT and pVNT among those who received it at a time interval of more than 6 weeks was 1.2 (95% CI: 1.1–1.3) and 1.8 (95% CI 1.2–2.7).
In Turkey, ( 27 ) et al. assessed the variables influencing the antibody response in 235 adults over 65 years of age following two doses of the inactivated SARS-CoV-2 vaccination (CoronaVac). Four weeks following the first and second vaccination doses, the mean levels of anti-SARS-CoV-2 IgG antibodies were 37.70 ± 57.08 IU/mL and 194.61 ± 174.88 IU/mL, respectively. Additionally, 4 weeks following the first vaccination dose, 134 out of 235 participants (57.02%) had an antibody level of less than 25.6 IU/mL (negative); 4 weeks following the second vaccination dose, this percentage was 11.48% ( n = 27). Eight participants (29.6%) had no comorbidities, while 19 (70.4%) with an antibody level less than 25.6 IU/mL 4 weeks after the first dose of the vaccination had at least one comorbid condition, including diabetes mellitus ( F = 2.352, p = 0.006). Individuals with comorbidities and those 65 years of age or older showed lower antibody response rates.
Demirbakan et al. ( 28 ) examined the presence of immunoglobulin G antibodies in the receptor-binding region of the S1 subunit of the SARS-CoV-2 spike protein in 1072 healthcare workers following immunization in a descriptive observational research. 28 days, 21 days, and 3 months following the first, second, and second dosages, respectively, were the times at which blood samples were taken. Anti-spike antibodies were found in 834/1072 (77.8%) subjects 4 weeks following the initial vaccination dose. Between 18 and 34 years of age, seropositivity was observed to be greater in both men and women (84.6%) compared to 70.6% ( p < 0.001) in the former group. In 1008 of 1,012 (99.6%) cases, anti-spike antibodies were found 21 days after the second dose, and in 803 of 836 (96.1%) cases, anti-spike antibodies were found 3 months later.
3.2.2.4 Safety
According to Bueno et al. ( 14 ) in their placebo-controlled clinical trial, pain at the injection site was the primary adverse reaction in 434 volunteers, and it occurred more frequently in the vaccine arm than in the placebo arm. The majority of the negative effects that were seen were modest and limited. No significant negative events were noted.
The frequency of adverse reactions was reported by Zeng et al. ( 25 ) without providing any additional effect measurements. In every immunization group, all adverse responses that were reported within 28 days after the third dose were classified as either grade 1 or 2. In the 1a-14d-2 m cohort, 150 participants reported three serious adverse events (2%); in the 1b-14d-8 m cohort, 150 participants reported four (3%); in the 2a-28d-2 m and 2b-28d-8 m cohorts, 150 participants reported one (1%); overall 349 people reported 24 (7%) serious adverse events.
Cheng et al. ( 18 ) observed an incidence rate of any adverse events of special interest following the first vaccination dose of 34.28 (95% CI: 29.81–39.23) and 38.39 (95% CI: 34.81–42.23) per 10,000 doses of BNT162b2 and CoronaVac, respectively, in their retrospective cohort of patients with chronic kidney disease. BNT162b2 (incidence rate ratio [95% CI]: first dose: 0.86 [0.69–1.08]; second dose: 0.96 [0.76–1.22]; third dose: 0.60 [0.33–1.10]) and CoronaVac (incidence rate ratio [95% CI]: first dose: 0.76 [0.64–0.91]; second dose: 0.86 [0.71–1.05]; third dose: 0.74 [0.36–1.54]) did not show an increased risk of overall adverse event of special interest when compared to the baseline period.
4 Discussion
The COVID-19 pandemic has affected the world’s population with a high morbidity and mortality rate. Recent reports have described persistent symptoms that extend beyond the initial period of the disease. It has been observed that adverse consequences, in addition to respiratory effects, are produced at different levels: cardiovascular, neurological, or immunological; cutaneous, gastrointestinal, or kidney manifestations, as well as in mental health, both as a result of acute infection and by the so-called post-COVID-19 syndrome ( 29 ). In this context, developing effective and safe vaccines was the determining control measure for pandemic management since, in addition to reducing the transmission of infections and allowing the control of the disease, vaccines had a determining role in reducing severe and fatal complications associated with infection ( 30 ). In addition to the above, the time in which the vaccine candidates were available, where it took less than a year for developers to complete the design, manufacturing, efficacy and safety testing and evaluation and approval for use, is an immeasurable scientific and public health learning, as well as an example of cooperation between healthcare authorities, the scientific community and private sector ( 31 ).
This review presents an analysis of the methods, populations, and scope of the studies that have evaluated the efficacy/effectiveness and safety of the vaccines available for COVID-19, emphasizing CoronaVac. Differences were found in terms of the proportion of populations evaluated, follow-up times, and times of the studies regarding the appearance of variants of concern.
Although some clinical trials with random assignment have been carried out to assess efficiency and safety outcomes with CoronaVac, these have limitations in terms of feasibility, follow-up times, and with this, the possibility of evaluating safety outcomes that occur with low frequencies ( 32 ). In this sense, it is important to carry out observational data analysis. However, not all studies have used homogeneous methods of analysis. Both the prevention of infection, and the prevention of outcomes such as hospitalization or death, have been valued through similar outcomes, but some through multivariate analysis of dependencies, and others through analysis that try to infer causally through different control methods of confounding. Studies have compared the evaluation of the same outcome through different methods, including multivariable logistic regression, propensity matching, propensity adjustment, and propensity-base weighting. However, researchers described that the estimates are very sensitive to the explicit or implicit weighting system in an adjustment technique, so it must be clear for which population a global treatment estimate is most appropriate ( 33 ).
It is important to recognize that there are common challenges in the collection, notification, and use of epidemiological data, such as the exhaustiveness and representativeness of the results and their comparability in time, among others. Therefore, it is necessary to identify the strongest analytical designs (among them the interrupted temporal series and comparative longitudinal studies), accompanied by sensitivity analysis of the results and being explicit, starting from the design, in the type of biases and problems that can be found in the data analysis that is available ( 34 ).
Concerning the evaluation of the immune response to the different types of vaccines, it has been oriented both to the antibody-mediated response and that mediated by cellular immunity. Among the antibody-mediated response, the reference standard has been established with the specific neutralizing antibody response against spike proteins of the virus, and a proxy to this response assessing neutralizing capacity has been measured in other studies by immunoglobulin G (IgG) antibody levels against the SARS-CoV-2 receptor binding domain (RBD) ( 35 ).
In the different studies, the decrease in the response levels to specific neutralizing antibodies was assumed to indicate the vaccine protection level when the levels of specific neutralizing antibodies fell between 4 and 6 months. The statistical methods used for their measurement are not homogeneous among all studies which has been used to recommend the application of boosters with vaccines produced in homologous or heterologous platforms of those received in established vaccination schemes ( 36 , 37 ).
To assess the duration of vaccine protection in the real world, it is also important to consider the difficulties in assessing the cellular memory immune response. The measurement of the CD4+ and CD8+ T lymphocytes response expressed in the production of different activation markers is heterogeneous, depending on antigenic stimuli such as peptides from circulating virus variants, cells from infected individuals, or peptides from different vaccines, in addition to diversity in the host response, which does not allow to have precise indicators to define optimal vaccination schedules ( 38 , 39 ).
In this context, inactivated whole virus vaccines, such as CoronaVac, by preserving epitopes of the virus, could respond in a broader spectrum to the different variants of circulating viruses or to new mutations, which could lead to the optimization of global vaccination schedules ( 10 ).
The main strength of our study lies in its systematic development, which reduces the possibility of biases in study selection. The use of different databases, including Latin American ones, allows for a broader search, although it is acknowledged that due to the magnitude of research on this topic, there may still be unreported or unfound studies, behaving as gray literature. The review results enabled us to achieve our objective, which was to describe how the efficacy/effectiveness of COVID-19 vaccines has been evaluated, with emphasis on CoronaVac. This allowed for the identification of some differences in these methods and some persisting gaps in defining more homogeneous methods for evaluation, regardless of whether these studies had high or low certainty in their evidence, which should be revisited if the objective is to evaluate the effectiveness and/or safety in the population of these interventions. However, the findings presented could be assessed and discussed with broader groups of experts in the field, which would help generate more accurate recommendations regarding their significance and potential implications.
In addition to the mentioned limitations, it is important to acknowledge that this type of review, having less precise question definitions compared to systematic reviews of effectiveness and safety (with their PICO structure), may result in some gaps in the application of search terms that could affect the results. Additionally, the vast amount of information, as was the case in our review, can create difficulties in synthesis and analysis, so it is crucial, as mentioned, to continue the discussion in groups with increasingly greater expertise in the subject ( 40 ). Lastly, while it is tempting to provide quantitative results regarding the synthesis conducted, the most important aspect is to address the original question regarding the gaps in the evaluation of these vaccines.
5 Conclusion
Published information on the evaluation of the efficacy/effectiveness and safety of the different vaccines against COVID-19 is abundant. However, there are differences in terms of vaccine application schedules, population definition, outcomes evaluated, follow-up times, and safety assessment, as well as non-standardization in the reporting of results, which may hinder the generalizability of the findings. It is important to define the relevance of the analysis methods in advance, considering these differences and the heterogeneity that can be produced in the analysis and meta-analysis of this information. It is important to generate meetings and consensus strategies for the methods and reporting of this type of studies, which will allow to reduce the heterogeneity in their presentation and a better understanding of the effect of these vaccines.
Data availability statement
The original contributions presented in the study are included in the article/ Supplementary material , further inquiries can be directed to the corresponding author.
Author contributions
JA-Á: Conceptualization, Methodology, Writing – original draft, Writing – review & editing, Investigation. PA-V: Writing – original draft, Writing – review & editing, Investigation. DA-L: Writing – original draft, Writing – review & editing, Investigation. AC-E: Writing – original draft, Writing – review & editing, Investigation. AG-H: Writing – original draft, Writing – review & editing, Investigation. LL-C: Writing – original draft, Writing – review & editing, Investigation. IM: Writing – original draft, Writing – review & editing, Investigation. DO-L: Writing – original draft, Writing – review & editing, Investigation. GQ: Writing – original draft, Writing – review & editing, Investigation. SR-B: Writing – original draft, Writing – review & editing, Investigation. CR-B: Funding acquisition, Writing – original draft, Writing – review & editing, Investigation. PR: Writing – original draft, Writing – review & editing, Investigation. MS-O: Writing – original draft, Writing – review & editing, Investigation. CT-A: Writing – original draft, Writing – review & editing, Investigation. MA-M: Funding acquisition, Project administration, Writing – original draft, Writing – review & editing, Investigation, Project Administration.
The author(s) declare financial support was received for the research, authorship, and/or publication of this article. CDC-China COVEP Fund Project #211-CO-04 (Submission 03/06/2022). The funders had no role in the design and development of the study, collection, management, analysis, interpretation of the data, writing the report, and the decision to submit the manuscript for publication.
Acknowledgments
We would like to thank Doracelly Hincapié-Palacio and María Teresa Rugeles-López, professors and researchers at the University of Antioquia, for their contributions and suggestions in the discussion of the results.
Conflict of interest
The authors declare that the research was conducted in the absence of any commercial or financial relationships that could be construed as a potential conflict of interest.
Publisher’s note
All claims expressed in this article are solely those of the authors and do not necessarily represent those of their affiliated organizations, or those of the publisher, the editors and the reviewers. Any product that may be evaluated in this article, or claim that may be made by its manufacturer, is not guaranteed or endorsed by the publisher.
Supplementary material
The Supplementary material for this article can be found online at: https://www.frontiersin.org/articles/10.3389/fpubh.2024.1321327/full#supplementary-material
1. Sharma, D, and Shalimar,. COVID-19: finally on wane, with reduced lethality. Comb Chem High Throughput Screen . (2022) 25:768–70. doi: 10.2174/1386207324666210811130046
PubMed Abstract | Crossref Full Text | Google Scholar
2. Hodgson, SH, Mansatta, K, Mallett, G, Harris, V, Emary, KRW, and Pollard, AJ. What defines an efficacious COVID-19 vaccine? A review of the challenges assessing the clinical efficacy of vaccines against SARS-CoV-2. Lancet Infect Dis . (2021) 21:e26–35. doi: 10.1016/S1473-3099(20)30773-8
3. Fang, E, Liu, X, Li, M, Zhang, Z, Song, L, Zhu, B, et al. Advances in COVID-19 mRNA vaccine development. Signal Transduct Target Ther . (2022) 7:94. doi: 10.1038/s41392-022-00950-y
4. Doherty, M, Buchy, P, Standaert, B, Giaquinto, C, and Prado-Cohrs, D. Vaccine impact: benefits for human health. Vaccine . (2016) 34:6707–14. doi: 10.1016/j.vaccine.2016.10.025
Crossref Full Text | Google Scholar
5. Conklin, L, Hviid, A, Orenstein, WA, Pollard, AJ, Wharton, M, and Zuber, P. Vaccine safety issues at the turn of the 21st century. BMJ Glob Health . (2021) 6:898. doi: 10.1136/bmjgh-2020-004898
6. Tregoning, JS, Flight, KE, Higham, SL, Wang, Z, and Pierce, BF. Progress of the COVID-19 vaccine effort: viruses, vaccines and variants versus efficacy, effectiveness and escape. Nat Rev Immunol . (2021) 21:626–36. doi: 10.1038/s41577-021-00592-1
7. Williams, LR, Ferguson, NM, Donnelly, CA, and Grassly, NC. Measuring vaccine efficacy against infection and disease in clinical trials: sources and magnitude of bias in coronavirus disease 2019 (COVID-19) vaccine efficacy estimates. Clin infect dis: official publication of the Infect Dis Society of America agosto de . (2022) 75:e764–73. doi: 10.1093/cid/ciab914
8. Fiolet, T, Kherabi, Y, MacDonald, CJ, Ghosn, J, and Peiffer-Smadja, N. Comparing COVID-19 vaccines for their characteristics, efficacy and effectiveness against SARS-CoV-2 and variants of concern: a narrative review. Clin microbiol infect: official publication of the European Society of Clin Microbiol Infect Dis . (2022) 28:202–21. doi: 10.1016/j.cmi.2021.10.005
9. Graña, C, Ghosn, L, Evrenoglou, T, Jarde, A, Minozzi, S, Bergman, H, et al. Efficacy and safety of COVID-19 vaccines. Cochrane Database Syst Rev . (2022) 2023:477. doi: 10.1002/14651858.CD015477
10. Jin, L, Li, Z, Zhang, X, Li, J, and Zhu, F. CoronaVac: a review of efficacy, safety, and immunogenicity of the inactivated vaccine against SARS-CoV-2. Hum Vaccin Immunother . (2022) 18:2096970. doi: 10.1080/21645515.2022.2096970
11. Peters, MDJ, Godfrey, C, McInerney, P, Munn, Z, Tricco, AC, and Khalil, H. Chapter 11: Scoping Reviews (2020 version). E Aromataris and Z Munn, editors. JBI Manual for Evidence Synthesis. JBI; (2020). Available from https://synthesismanual.jbi.global .
Google Scholar
12. Ouzzani, M, Hammady, H, Fedorowicz, Z, and Elmagarmid, A. Rayyan-a web and mobile app for systematic reviews. Systematic Rev . (2016) 5:210. doi: 10.1186/s13643-016-0384-4
13. Boruah, A, Westenberg, E, Hanif Khan, A, Kee Hoo, F, Guekht, A, Spatola, M, et al. Characterization and analysis of neurologic adverse events associated with COVID-19 vaccination. Neurol Int . (2022) 98:784. doi: 10.1212/WNL.98.18_supplement.3784
14. Bueno, SM, Abarca, K, González, PA, Gálvez, NMS, Soto, JA, Duarte, LF, et al. Safety and immunogenicity of an inactivated severe acute respiratory syndrome coronavirus 2 vaccine in a subgroup of healthy adults in Chile. Clin infect dis: official publication of the Infect Dis Society of America . (2022) 75:e792–804. doi: 10.1093/cid/ciab823
15. Duarte, LF, Gálvez, NMS, Iturriaga, C, Melo-González, F, Soto, JA, Schultz, BM, et al. Immune profile and clinical outcome of breakthrough cases after vaccination with an inactivated SARS-CoV-2 vaccine. Front Immunol . (2021) 12:742914. doi: 10.3389/fimmu.2021.742914
16. Tanriover, MD, Doğanay, HL, Akova, M, Güner, HR, Azap, A, Akhan, S, et al. Efficacy and safety of an inactivated whole-virion SARS-CoV-2 vaccine (CoronaVac): interim results of a double-blind, randomised, placebo-controlled, phase 3 trial in Turkey. Lancet (London, England) . (2021) 398:213–22. doi: 10.1016/S0140-6736(21)01429-X
17. Fadlyana, E, Rusmil, K, Tarigan, R, Rahmadi, AR, Prodjosoewojo, S, Sofiatin, Y, et al. A phase III, observer-blind, randomized, placebo-controlled study of the efficacy, safety, and immunogenicity of SARS-CoV-2 inactivated vaccine in healthy adults aged 18-59 years: an interim analysis in Indonesia. Vaccine . (2021) 39:6520–8. doi: 10.1016/j.vaccine.2021.09.052
18. Cheng, FWT, Fan, M, Wong, CKH, Chui, CSL, Lai, FTT, Li, X, et al. The effectiveness and safety of mRNA (BNT162b2) and inactivated (CoronaVac) COVID-19 vaccines among individuals with chronic kidney diseases. Kidney Int . (2022) 102:922–5. doi: 10.1016/j.kint.2022.07.018
19. Can, G, Acar, HC, Aydin, SN, Balkan, II, Karaali, R, Budak, B, et al. Waning effectiveness of CoronaVac in real life: a retrospective cohort study in health care workers. Vaccine . (2022) 40:2574–9. doi: 10.1016/j.vaccine.2022.03.032
20. Jara, A, Undurraga, EA, Zubizarreta, JR, González, C, Pizarro, A, Acevedo, J, et al. Effectiveness of homologous and heterologous booster doses for an inactivated SARS-CoV-2 vaccine: a large-scale prospective cohort study. Lancet Glob Health . (2022) 10:e798–806. doi: 10.1016/S2214-109X(22)00112-7
21. Cerqueira-Silva, T, Andrews, JR, Boaventura, VS, Ranzani, OT, de Araújo, OV, Paixão, ES, et al. Effectiveness of CoronaVac, ChAdOx1 nCoV-19, BNT162b2, and Ad26.COV2.S among individuals with previous SARS-CoV-2 infection in Brazil: a test-negative, case-control study. The lancet. Infect Dis Ther . (2022) 22:791–801. doi: 10.1016/S1473-3099(22)00140-2
22. Cerqueira-Silva, T, Katikireddi, SV, de Araujo, OV, Flores-Ortiz, R, Júnior, JB, Paixão, ES, et al. Vaccine effectiveness of heterologous CoronaVac plus BNT162b2 in Brazil. Nat Med . (2022) 28:838–43. doi: 10.1038/s41591-022-01701-w
23. Ranzani, OT, Hitchings, MDT, de Melo, RL, de França, GVA, de FR, FC, Lind, ML, et al. Effectiveness of an inactivated Covid-19 vaccine with homologous and heterologous boosters against Omicron in Brazil. Nat Commun . (2022) 13:5536. doi: 10.1038/s41467-022-33169-0
24. Sritipsukho, P, Khawcharoenporn, T, Siribumrungwong, B, Damronglerd, P, Suwantarat, N, Satdhabudha, A, et al. Comparing real-life effectiveness of various COVID-19 vaccine regimens during the delta variant-dominant pandemic: a test-negative case-control study. Emerg Microbes Infect . (2022) 11:585–92. doi: 10.1080/22221751.2022.2037398
25. Zeng, G, Wu, Q, Pan, H, Li, M, Yang, J, Wang, L, et al. Immunogenicity and safety of a third dose of CoronaVac, and immune persistence of a two-dose schedule, in healthy adults: interim results from two single-Centre, double-blind, randomised, placebo-controlled phase 2 clinical trials. Lancet Infect Dis . (2022) 22:483–95. doi: 10.1016/S1473-3099(21)00681-2
26. Chantasrisawad, N, Puthanakit, T, Kornsitthikul, K, Jaru-Ampornpan, P, Tawan, M, Matapituk, P, et al. Immunogenicity to SARS-CoV-2 Omicron variant among school-aged children with 2-dose of inactivated SARS-CoV-2 vaccines followed by BNT162b2 booster. Vaccine: X . (2022) 12:100221. doi: 10.1016/j.jvacx.2022.100221
27. Karamese, M, and Tutuncu, EE. The effectiveness of inactivated SARS-CoV-2 vaccine (CoronaVac) on antibody response in participants aged 65 years and older. J Med Virol . (2022) 94:173–7. doi: 10.1002/jmv.27289
28. Demirbakan, H, Koçer, I, Erdoğan, M, and Bayram, A. Assessing humoral immune response after two doses of an inactivated SARS-CoV-2 vaccine (CoronaVac) in healthcare workers. Public Health . (2022) 205:1–5. doi: 10.1016/j.puhe.2022.01.011
29. Peramo-Álvarez, FP, López-Zúñiga, MÁ, and López-Ruz, MÁ. Medical sequels of COVID-19. Med Clin (Barc) . (2021) 157:388–94. doi: 10.1016/j.medcli.2021.04.023
30. Park, JW, Lagniton, PNP, Liu, Y, and Xu, RH. mRNA vaccines for COVID-19: what, why and how. Int J Biol Sci . (2021) 17:1446–60. doi: 10.7150/ijbs.59233
31. Hodgson, J . The pandemic pipeline. Nat Biotechnol . (2020) 38:523–32. doi: 10.1038/d41587-020-00005-z
32. Gilmartin-Thomas, JF, Liew, D, and Hopper, I. Observational studies and their utility for practice. Aust Prescr . (2018) 41:82–5. doi: 10.18773/austprescr.2018.017
33. Kurth, T, Walker, AM, Glynn, RJ, Chan, KA, Gaziano, JM, Berger, K, et al. Results of multivariable logistic regression, propensity matching, propensity adjustment, and propensity-based weighting under conditions of nonuniform effect. Am J Epidemiol . (2006) 163:262–70. doi: 10.1093/aje/kwj047
34. Stoto, MA, Woolverton, A, Kraemer, J, Barlow, P, and Clarke, M. COVID-19 data are messy: analytic methods for rigorous impact analyses with imperfect data. Glob Health . (2022) 18:2. doi: 10.1186/s12992-021-00795-0
35. Lopera, TJ, Chvatal-Medina, M, Flórez-Álvarez, L, Zapata-Cardona, MI, Taborda, NA, Rugeles, MT, et al. Humoral response to BNT162b2 vaccine against SARS-CoV-2 variants decays after six months. Front Immunol . (2022) 13:879036. doi: 10.3389/fimmu.2022.879036
36. Widge, AT, Rouphael, NG, Jackson, LA, Anderson, EJ, Roberts, PC, Makhene, M, et al. Durability of responses after SARS-CoV-2 mRNA-1273 vaccination. N Engl J Med . (2021) 384:80–2. doi: 10.1056/NEJMc2032195
37. Doria-Rose, N, Suthar, MS, Makowski, M, O’Connell, S, McDermott, AB, Flach, B, et al. Antibody persistence through 6 months after the second dose of mRNA-1273 vaccine for Covid-19. N Engl J Med . (2021) 384:2259–61. doi: 10.1056/NEJMc2103916
38. Primorac, D, Vrdoljak, K, Brlek, P, Pavelić, E, Molnar, V, Matišić, V, et al. Adaptive immune responses and immunity to SARS-CoV-2. Front Immunol . (2022) 13:848582. doi: 10.3389/fimmu.2022.848582
39. Benjamanukul, S, Traiyan, S, Yorsaeng, R, Vichaiwattana, P, Sudhinaraset, N, Wanlapakorn, N, et al. Safety and immunogenicity of inactivated COVID-19 vaccine in health care workers. J Med Virol . (2022) 94:1442–9. doi: 10.1002/jmv.27458
40. Mak, S, and Thomas, A. An introduction to scoping reviews. J Grad Med Educ . (2022) 14:561–4. doi: 10.4300/JGME-D-22-00620.1
Keywords: COVID-19, SARS-CoV-2, vaccines, CoronaVac, review
Citation: Alzate-Ángel JC, Avilés-Vergara PA, Arango-Londoño D, Concha-Eastman A, Garcés-Hurtado A, López-Carvajal L, Minotta IL, Ortega-Lenis D, Quintero G, Reina-Bolaños S, Reina-Bolaños CA, Roa P, Sánchez-Orozco M, Tovar-Acero C and Arbeláez-Montoya MP (2024) How has research on the effectiveness and safety of COVID-19 vaccination been evaluated: a scope review with emphasis on CoronaVac. Front. Public Health . 12:1321327. doi: 10.3389/fpubh.2024.1321327
Received: 13 October 2023; Accepted: 25 March 2024; Published: 10 April 2024.
Reviewed by:
Copyright © 2024 Alzate-Ángel, Avilés-Vergara, Arango-Londoño, Concha-Eastman, Garcés-Hurtado, López-Carvajal, Minotta, Ortega-Lenis, Quintero, Reina-Bolaños, Reina-Bolaños, Roa, Sánchez-Orozco, Tovar-Acero and Arbeláez-Montoya. This is an open-access article distributed under the terms of the Creative Commons Attribution License (CC BY) . The use, distribution or reproduction in other forums is permitted, provided the original author(s) and the copyright owner(s) are credited and that the original publication in this journal is cited, in accordance with accepted academic practice. No use, distribution or reproduction is permitted which does not comply with these terms.
*Correspondence: Juan C. Alzate-Ángel, [email protected]
Disclaimer: All claims expressed in this article are solely those of the authors and do not necessarily represent those of their affiliated organizations, or those of the publisher, the editors and the reviewers. Any product that may be evaluated in this article or claim that may be made by its manufacturer is not guaranteed or endorsed by the publisher.
Evidence Review of the Adverse Effects of COVID-19 Vaccination and Intramuscular Vaccine Administration
Vaccines are a public health success story, as they have prevented or lessened the effects of many infectious diseases. To address concerns around potential vaccine injuries, the Health Resources and Services Administration (HRSA) administers the Vaccine Injury Compensation Program (VICP) and the Countermeasures Injury Compensation Program (CICP), which provide compensation to those who assert that they were injured by routine vaccines or medical countermeasures, respectively. The National Academies of Sciences, Engineering, and Medicine have contributed to the scientific basis for VICP compensation decisions for decades.
HRSA asked the National Academies to convene an expert committee to review the epidemiological, clinical, and biological evidence about the relationship between COVID-19 vaccines and specific adverse events, as well as intramuscular administration of vaccines and shoulder injuries. This report outlines the committee findings and conclusions.
Read Full Description
- Digital Resource: Evidence Review of the Adverse Effects of COVID-19 Vaccination
- Digital Resource: Evidence Review of Shoulder Injuries from Intramuscular Administration of Vaccines
Recent News
Science Academies Issue Statements to Inform G7 Talks
Supporting Family Caregivers in STEMM
A Vision for High-Quality Preschool for All
Pregnant and Lactating Women in Clinical Research
- Load More...

An official website of the United States government
The .gov means it’s official. Federal government websites often end in .gov or .mil. Before sharing sensitive information, make sure you’re on a federal government site.
The site is secure. The https:// ensures that you are connecting to the official website and that any information you provide is encrypted and transmitted securely.
- Publications
- Account settings
Preview improvements coming to the PMC website in October 2024. Learn More or Try it out now .
- Advanced Search
- Journal List
- Wiley - PMC COVID-19 Collection

COVID‐19 vaccine research and development: ethical issues
1 Department of Microbiology, Faculty of Medicine Public Health and Nursing, Universitas Gadjah Mada, Yogyakarta Indonesia
2 Medical and Health Research Ethics Committee, Faculty of Medicine Public Health and Nursing, Universitas Gadjah Mada / Dr. Sardjito General Hospital, Yogyakarta Indonesia
The achievements of vaccine research and development bring a hope to our societies that we may cope with the COVID‐19 pandemic. There are two aspects that should be maintained in balance: the immediate necessity for speed of vaccine research and the inherent need for protection of research subjects, which is the foremost concern of research ethics. This narrative review highlights ethical issues in COVID‐19 vaccine research and development that every stakeholder needs to be aware of and to consider.
Introduction
COVID‐19 is a deadly disease which continues to affect many countries in the world. The incidence is higher in the Americas (14 117 714 cases and 486 843 deaths) and Europe (4 515 514 cases and 222 624 deaths) than in South East Asia (4 786 594 cases and 84 541 deaths), Africa (1 088 093 cases and 23 101 deaths) and the Western Pacific (520 012 cases and 11 306 deaths) [ 1 ].
Vaccines are the most important public health measure to protect people from COVID‐19 worldwide, since SARS‐CoV‐2 is highly contagious and infects populations widely and globally [ 2 ]. Traditionally, vaccine development takes years, even decades: from about 40 years for polio to 5 years for Ebola,most vaccines took 15 years on average [ 3 , 4 ]. The trial process for vaccines consists of several steps which need to be conducted systematically and in a measurable stride. The length of this process is correlated with the nature of the vaccine itself, which is to protect healthy people from being infected by pathogens. Adverse events and deleterious effects will not be tolerated, vaccines are not the same as drugs that are consumed by the sick. The risk–benefit analysis for prescription drugs and vaccine administration is different.
The invention of a successful and widely available COVID‐19 vaccine will be a great leap forward for humankind, but there are several challenges to overcome: (1) a lack of understanding of the pathogenesis and the predictive role of vaccines in the clinical pathway of persons being infected by SARS‐CoV‐2 [ 5 , 6 , 7 ], (2) a huge disagreement among experts about how to determine the most immunogenic epitopes and antigens of SARS‐CoV‐2 [ 8 , 9 ], (3) the finding that antibody‐dependent enhancement (ADE) may contribute to the exaggeration of SARS‐CoV‐2 disease [ 10 , 11 ], (4) the lack of established animal models for COVID‐19 vaccine challenge testing, which raises the speculation of using controlled human infection (CHI) as a potential approach [ 3 ], and finally, (5) speculation that the duration of protection by immune response in natural infection is not long enough [ 12 ].
The race for COVID‐19 vaccine invention and development against the spread and catastrophic effects of the disease is real. WHO released a draft list of COVID‐19 candidate vaccines on 3 September 2020. At least 34 vaccine candidates are in clinical evaluation to date [ 13 ]. Several new technologies are used as COVID‐19 vaccine development platforms. Conventional techniques for the development of vaccines such as inactivated, inactivated with adjuvant and live attenuated are still being used. However, reversed vaccinology approaches are also being emplyed, such as a recombinant subunit vaccine, and a more advanced approach using vector delivery systems, along with RNA‐ and DNA‐based vaccines (Table 1 ) [ 4 , 9 , 13 ].
Candidate COVID‐19 Vaccines in Clinical Trial Phases*
The attempts to accelerate vaccine development are associated with efforts to streamline the process. Unfortunately, streamlining may have consequences for the traditional ethics of vaccine research and development, especially the long‐held principles of beneficence and non‐maleficence. This short narrative review summarises the ethical issues that may emerge from the current directions in COVID‐19 vaccine research and development during the pandemic.
Vaccine candidates must fulfil several requirements: safety, efficacy and quality. Because of the current escalation of the global COVID‐19 pandemic, some aspects may change. The speed of vaccine development may push public health ministers, heads of states and the pharmaceutical industry to change their strategy for bulk budget investment for vaccine research. They must decide to prepare mass production events based on the limited data of promising vaccine candidates [ 14 ]. The need to protect billions of earth’s inhabitants pushes governments and societies of the world to a ‘great expectation’ for the new vaccine. The overriding expectation, although with diverse interests, may influence the objective judgement typically required of candidate vaccine safety. Protecting human lives should be the priority.
mRNA‐ [ 15 ] and DNA‐based vaccine technologies [ 9 , 16 ] are being implemented in humans, especially as vaccine candidates. Several concerns about mRNA vaccine safety have been identified besides its promising potential advantages. The most important risks include the possibility that mRNA vaccines may generate strong type I interferon responses that could lead to inflammation and autoimmune conditions [ 17 ]. The safety concerns of DNA‐based vaccines involve the possibility that the targeting of DNA into the chromosomal DNA of the acceptor will trigger mutagenic effects in the functional gene located in the insertion loci [ 18 ]. At present, there are no mRNA‐ and DNA‐based vaccines against any disease authorised to be marketed.
The strategy of DNA vaccines is similar to gene therapy in that a delivery system, such as plasmid, delivers targeted DNA into cells, where it is translated into proteins that induce the acceptors’ immune response to generate targeted T‐cell and antibody responses [ 19 ]. We have experience in using DNA for several gene therapies mostly related to inherited diseases or familial predispositions. Mainstream gene therapy scientists have stated that gene therapy is only suitable for terminally ill patients because the risks are very high [ 20 ]. Vaccine administration is completely different from interventions with gene therapy since the vaccine is for healthy human subjects, and the risk–benefit consideration would be completely different too. Both terminally ill and healthy persons have the same risk for the introduction of foreign DNA into their body, but terminally ill persons may benefit through having a chance to recover from their deadly disease, whereas healthy individuals may not have any benefit because they have never encountered the particular pathogen.
When we perform the risk assessment of new technology, it is based on a theoretical framework without direct evidence concerning to what extent the probability of the risk may occur. Theoretically, DNA vaccine may be able to induce autoimmune diseases and can be inserted into any part of the chromosomes [ 21 ]. Scientists know how the mechanism works and are able to predict the risk if it might happen. But nobody knows for certain how great the probability is of producing mutagenic and deleterious effects in one part of a gene sequence when inserted into another. For example, when a test subject named Jessie Gelsinger was injected with adeno‐associated viruses (AAVs), nobody expected the deadly risk that ultimately occurred in this research subject [ 22 ]. Accordingly, the risk–benefit assessment in the use of new technology should be done carefully. It is true that sometimes we have to deal with a risk possibility that is not immediately present but theoretically possible, and vice versa. Mitigation to the deleterious effect could be started prior to the clinical trial. However, there is always the possible existence of risks that have not been identified yet and will only show in the later phases of clinical trials.
In the current pandemic, all societies expect a breakthrough in medical and health technology. In a situation where understanding of the new disease is poor and no satisfactory medical technology is available for prevention and treatment yet, it is natural to think that ‘doing something is better than nothing’. This is going to make safety judgement among stakeholders more prone to deterioration.
Controlled human infection (CHI)
One of the crucial steps of vaccine development is the challenge test, which is used to measure the potential protection of the candidate. The challenge test is usually part of the pre‐clinical study in an animal model. However, in the case of COVID‐19 and some other diseases, an animal model is not available, although there are candidates that need to be verified [ 3 , 23 , 24 , 25 ]. It seems the pathogen does not produce a similar clinical course in common animal models, which excludes safety and efficacy data from animal models alone. There was a proposal of human challenge testing to replace the pre‐clinical challenge test in animal models, with the use of controlled human infection (CHI). It will solve the problem of the animal models’ unreliability and gain time for the developers especially in phase III [ 3 , 26 ].
To some extent, it is possible to perform these challenge tests with human volunteers. It sounds like an unsafe experimentation, but the choices are extremely limited. The next question is how can we do this experiment with the current ethical review process? The WHO has issued a guideline for CHI [ 27 ]. The guideline is broad and needs local ethics committee approval for its implementation. Considerations of the pros and cons of CHI are widely discussed in COVID‐19 vaccine development. Previously, CHI was used to develop vaccines against malaria [ 28 ], typhoid [ 29 ] and cholera [ 30 ], which are diseases with established treatment [ 31 ]. Subjects who suffered from deleterious effects after experimentation could be rescued by the established treatment. Application of CHI in COVID‐19 is a very different story because there is no standard treatment for this new and highly contagious disease. Nevertheless, there have been thousands of volunteers from 162 countries who declared their willingness to be participants in this CHI [ 32 ]. The need for a vaccine is prevalent in people’s minds and equally necessary from the public health point of view. Without any precedents, it is going to be difficult to judge the risks benefits in this matter [ 33 ].
Controlled human infection could be done in a situation where there is an attenuated virus strain available, for example, using an artificial mutant virus. This approach is to prevent fatal outcomes in trial subjects. But the challenge test results from attenuated virus may not be generalisable – the attenuated strain may not be similar enough to the naturally circulating virus. In addition, producing the attenuated virus may require another step that will take almost as much time to perform as the regular phase III in typical controlled clinical trials. This additional step in an already complicated process will render futile the main purpose to gain more time to develop an effective vaccine [ 34 ].
Location and population
Development sites of COVID‐19 vaccines are involving research subjects from many countries, for example USA, Russia, Argentina, Brazil, Germany, India, Saudi Arabia, Pakistan and others [ 35 ]. The need of multi‐centred research is obvious in the vaccine development. The safety, tolerability, and efficacy of the vaccines should be obtained from different geographic areas, ethnicities, prevalence and varieties of the virus circulating in the areas [ 36 ]. The attempt to fulfil this requirement may result in the involvement of countries with limited resources and whose underdeveloped infrastructure would make the people involved become even more vulnerable as research subjects from the ethical and humane point of view. The possible exploitation of vulnerable people from less developed countries should be reviewed thoroughly. The vaccine trial should give them equitable advantages in trade, such as capacity building, transfer of technology and access to the vaccine during the current pandemic of COVID‐19.
Another concern is the availability of an adequate health facility and system to ensure that trial subjects and their families and/or communities have access to treatment and proper care in case of serious adverse events related to the trial outcomes. This must be assessed before any clinical trials begin. Providing the most comprehensive health services to the trial population will be an added value for population involvement in the trial. The best practice of vaccine clinical trials should have direct benefits for the community, such as improvement and availability of basic health facilities [ 37 ]
Vaccine acceptors are sometimes segmented into target groups, which is related to the host distribution of the target disease, for example by gender, age and specific population in the endemic area. A vaccine clinical trial is usually started in adult subjects and continued to more vulnerable subjects such as infants, young children, the elderly and women. Clinical vaccine trials will recruit vulnerable subjects. Protection measures to safeguard the vulnerable and marginalised populations should be carefully scrutinised during review. Ethical considerations must be adjusted to the individual situation to protect these vulnerable subjects from exploitation and later abandonment [ 38 ].
However, in an emergency pandemic situation, the definition of vulnerability needs to be openly discussed, and emergency calls for exceptions. The exclusion of vulnerable groups may diminish trial validity because of selection bias, so they should not be excluded without reasonable scientific and ethical justification [ 39 ].
Post‐trial access
After clinical vaccine trials, the subjects should have access to the developed vaccine. This is part of their direct advantage for their involvement in the research. While it is mentioned in the international ethical guidelines, not all researchers know and are aware of this important obligation [ 40 ]. The current COVID‐19 vaccine development involves multi‐country and intercontinental research recruiting subjects from different countries and regions. The post‐trial access to COVID‐19 vaccines should be expanded beyond the community where the trial is performed to include the country and region.
Post‐trial access is a matter which must be addressed from the very beginning of research design. Community engagement should be considered prior to the trial and involve all stakeholders: sponsors, industries, developers, investigators, subjects of the trial, communities and the government where the trial is performed.
In summary, the current COVID‐19 vaccine research and development involves people from many countries, which raises ethical issues that must be addressed by all stakeholders. Even in the emergency of a pandemic, the urgency of providing an effective COVID‐19 vaccine for humankind must be balanced with the exigency of research ethics that must be maintained. In any event, the safety and well‐being of research subjects must be protected, especially that of vulnerable subjects.
Sustainable Development Goals (SDGs): SDG 3 (good health and well‐being)
Thank you for visiting nature.com. You are using a browser version with limited support for CSS. To obtain the best experience, we recommend you use a more up to date browser (or turn off compatibility mode in Internet Explorer). In the meantime, to ensure continued support, we are displaying the site without styles and JavaScript.
- View all journals
- My Account Login
- Explore content
- About the journal
- Publish with us
- Sign up for alerts
- Open access
- Published: 13 April 2024
Identifying and overcoming COVID-19 vaccination impediments using Bayesian data mining techniques
- Bowen Lei 1 ,
- Arvind Mahajan 2 &
- Bani Mallick 1
Scientific Reports volume 14 , Article number: 8595 ( 2024 ) Cite this article
- Data mining
- Health care economics
- Health policy
- Statistical methods
The COVID-19 pandemic has profoundly reshaped human life. The development of COVID-19 vaccines has offered a semblance of normalcy. However, obstacles to vaccination have led to substantial loss of life and economic burdens. In this study, we analyze data from a prominent health insurance provider in the United States to uncover the underlying reasons behind the inability, refusal, or hesitancy to receive vaccinations. Our research proposes a methodology for pinpointing affected population groups and suggests strategies to mitigate vaccination barriers and hesitations. Furthermore, we estimate potential cost savings resulting from the implementation of these strategies. To achieve our objectives, we employed Bayesian data mining methods to streamline data dimensions and identify significant variables (features) influencing vaccination decisions. Comparative analysis reveals that the Bayesian method outperforms cutting-edge alternatives, demonstrating superior performance.
Similar content being viewed by others
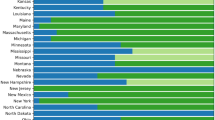
Association mining based approach to analyze COVID-19 response and case growth in the United States
Satya Katragadda, Raju Gottumukkala, … Ziad Ashkar
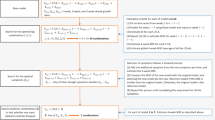
Short-term local predictions of COVID-19 in the United Kingdom using dynamic supervised machine learning algorithms
Xin Wang, Yijia Dong, … You Li
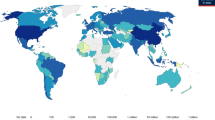
A global database of COVID-19 vaccinations
Edouard Mathieu, Hannah Ritchie, … Lucas Rodés-Guirao
Introduction
The emergence of COVID-19 has greatly impacted people’s lives since 2020 and will continue to do so. The Center for Systems Science and Engineering (CSSE) at Johns Hopkins University 1 reports that there have been more than 676 million cases and 6.8 million deaths in the world. To combat COVID-19, there are a number of restrictive methods to inhibit the spread of the virus 2 , 3 , 4 , 5 . These include lockdowns, quarantine, etc. These methods are widely used in many countries but many studies raise concerns about the costs and side effects of their use 6 , 7 , 8 , 9 , 10 , such as loss of gross domestic product (GDP), educational opportunities, increased deaths, higher mental health risks, and other societal costs. In addition to these restrictive methods, vaccines are another potent way to tackle the pandemic 3 , 11 , 12 . Higher vaccination rates would bring many benefits. However, the facts show that many people are unable or hesitant to get vaccinated 12 , 13 , 14 , 15 , 16 , 17 , 18 , 19 . In our study, impediments to COVID-19 vaccination are defined as unwillingness or refusal to receive the COVID-19 vaccine, or inability to receive the COVID-19 vaccine due to lack of vaccine availability ( CDC provides a definition of vaccination hesitancy measure at the following link https://data.cdc.gov/stories/s/Vaccine-Hesitancy-for-COVID-19/cnd2-a6zw/ . However, this is a subset of our impediment measure since CDC hesitancy measure doesn’t consider the lack of availability.) We aim to predict vaccine impediment using Bayesian technique and to identify groups of important variables that contribute to impediments to vaccination. We then make policy recommendations to address impediments to vaccination. The World Health Organization (WHO) has recognized vaccine impediment as one of the top ten global health threats, as it can lead to low vaccination rates and the resurgence of preventable diseases. This impediment can stem from a variety of reasons.
In this paper, we conducted an analysis of data sourced from a prominent health insurance provider in the United States. We briefly present how the vaccine impediment varies across insured populations, including gender, race, income level, and age, as shown in Fig. 1 . In terms of gender, similar to results of previous research 20 , we can see that women and men have almost the same percentage of vaccine impediments, with males having slightly higher impediments to vaccination. For different racial groups, Whites and Asians get relatively low impediment scores, while Hispanics, Blacks, and Native Americans have higher scores based on the data. A similar pattern has been found in existing works 21 . For each income level, people tend to be more willing to vaccinate as their income increases, from the lower to the upper middle class, which is also found in other studies 22 . However, the upper class is similar to the lower class, who are hampered in terms of vaccination. For different age groups, young and middle-aged people (from 20 to 50 years old) have very similar rates and are more hesitant to be vaccinated. In contrast, older people are more willing to vaccinate and the willingness increases with age (from 51 years and older). This is consistent with the findings of existing studies 22 , 23 , 24 .
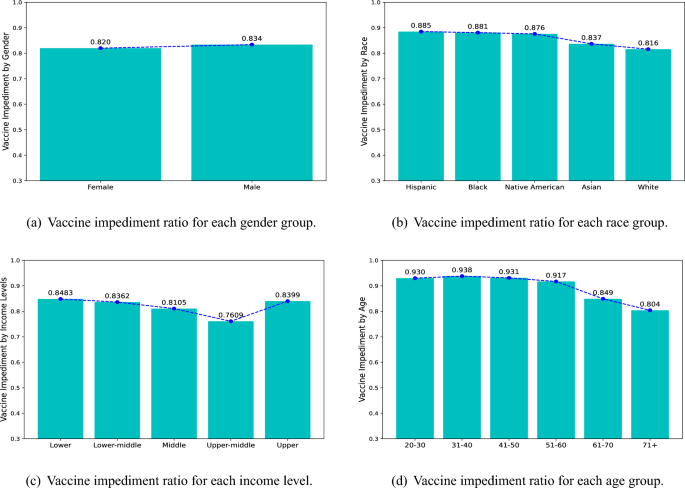
Vaccine impediment grouped by variables ( a ) gender, ( b ) race, ( c ) income level, and ( d ) age.
Impediments to vaccination are influenced by a variety of factors, and our goal was to gain deeper insights into the obstacles preventing individuals from getting vaccinated, identify them at an early stage, and formulate data-driven policies to address these challenges. This paper makes significant contributions to the existing literature in two key aspects. Firstly, it leverages granular and objective data obtained from a major health insurance provider, enabling a more in-depth and comprehensive analysis. Secondly, we employ an advanced classification model to predict the likelihood of a member being hesitant to receive the vaccine, yielding more accurate results compared to other statistical methods. Although we have used COVID-19 vaccination data, most of the results will likely be applicable to other epidemic or pandemic vaccination situations.
In this study, we introduce a two-stage methodology. In the first stage, we employ Bayes factor 25 , 26 for preliminary screening, followed by the application of a Bayesian nonparametric regression technique known as Bayesian Multivariate Adaptive Regression Splines (BMARS) 27 , 28 , 29 in the second stage. This approach is applied to population characteristic data provided by a major health insurance provider, with the aim of identifying barriers to vaccination. The pre-screening step enables our approach to effectively handle high-dimensional feature spaces by selecting the key features, simplifying the complex problem within the Bayesian framework. Additionally, the BMARS regression method allows for the modeling of nonlinear relationships between these selected key features and the response variable.
In the following sections, we first describe our Bayes-factor-based pre-screening and BMARS-based classification modeling (B-BMARS) method and introduce the vaccine impediment dataset to identify vaccination impediments. We then compare the results of B-BMARS with other popular baseline methods and analyze which variables play a key role in impeding getting vaccinated. Next, based on the modeling results, we present analyses and policy implications from the business perspective. We also describe other alternative baseline forecasting methods in the Supplementary Information.
We propose a novel two-stage method to accurately and efficiently analyze people’s impediments to receiving the COVID-19 vaccine with proper selections of interpretable variables and their interactions. The first stage, pre-screening, is based on the Bayes factor, a widely used Bayesian method to quickly check the correlation between variables and response. Thus, we can effectively filter out apparently irrelevant variables and avoid unnecessary computational burdens and modeling challenges. In the second stage of BMARS-based classification, the unknown function is fitted by product-based spline basis functions, which can automatically fine-tune the selection of key variables and their interactions.
Stage I: Bayes-factor-based pre-screening
In our COVID-19 vaccination data analysis, the dimension of potential key variables is usually too high to use Bayesian nonparametric models directly. Therefore, it is necessary to reduce the dimensionality of the variable space. We propose to take advantage of the model comparison ability of the Bayes factor and use it as a screening step to reduce the dimensions. Since our goal is to predict vaccine impediments, it becomes a binary classification problem. Therefore, we chose a method widely used for classification tasks, the Probit model, in which the conditional probability of one of the two possible attitudes toward the vaccine is equal to a linear combination of the underlying variables, transformed by the cumulative distribution function of the standard Gaussian 30 , 31 . For classification tasks, a widely used approach is to combine the regression model with a probit model using auxiliary variables. Specifically, in the classification framework, we use z to denote the observed response, which is a binary variable and y as the auxiliary variable. We assume the binary z to be 1 if \(y>0\) and 0 otherwise. For the probabilistic model, it is defined as \(p(z=1|y)=\Phi (y)\) where \(\Phi\) is the standard Gaussian cumulative distribution function and y is defined as \(y \sim \mathcal {N}(\varvec{ \beta }{\textbf {x}}+\beta _0, \sigma ^2)\) where \(\varvec{ x}\) is the \(p^*\) dimensional explanatory variables (covariates), \(\varvec{ \beta }\) is the vector of regression parameters and \(\sigma ^2\) is the error variance.
High-dimensional data analysis is always a daunting task. When the dimension \(p^*\) is high, we run into a problem called “the curse of dimensionality” 32 . Though the high dimensional variables usually provide more information, they also lead to higher computational costs. The convergence of optimization algorithms or Bayesian sampling in a space of high dimensions is usually very slow. Also, it can harm the estimation accuracy, which is due to the difficult search in a space of high dimensions. Therefore, an effective and accurate variable selection is essential in high-dimensional modeling.
Pre-screening is a popular way to quickly filter out unimportant variables, making variable selection more efficient in a much lower-dimension space using a simpler model (like linear model), especially for ultrahigh-dimensional cases. In pre-screening methods, it is usually assumed that if one variable is important when predicting the response, it will be marginally associated with the response. Different measurements of the association are studied using, for example, p-value 32 , 33 , 34 . However, the pre-screening technique have not been fully explored in the Bayesian paradigm.
We use an off-the-shelf Bayesian method, Bayes factor 35 , 36 , for pre-screening. More specifically, the Bayes factor is a Bayesian alternative to classical hypothesis testing, which plays an important role in the model comparison and selection process. Essentially, the Bayes factor serves as a measure of how strongly data support a specific model compared to another. The Bayes factor is defined as a ratio of the marginal likelihood of two candidate models, typically regarded as a null and an alternative hypothesis. The general formula is as below.
where D denotes the available data and \(M_1\) and \(M_2\) denote two potential models. A larger value of this ratio indicates more support for \(M_1\) , and vice versa.
More specifically, to check the effect of the j th variable \(x_{j}\) with the corresponding regression parameter \(\beta _{j}\) , we calculate the Bayes factor ( \(\text {BF}_j\) ) via Probit regression model as below
where hypothesis \(\mathscr {H}_1\) assumes that \(y \sim \mathcal {N}(\beta _j x_j+\beta _0, \sigma _{j}^2)\) , hypothesis \(\mathscr {H}_0\) assumes that \(y \sim \mathcal {N}(\beta _0, \sigma ^2)\) , prior for \(\beta _j\) is Gaussian distribution \(p(\beta _j)\sim \mathcal {N}(0,\alpha )\) , and use conjugate prior for the variances.
To compute the intractable marginal likelihood \(p({\textbf {z}} | \mathscr {H}_1)\) (integrated over \(\varvec{ \beta }\) ), we choose to use Laplace Approximation 37 , 38 , 39 . Specifically, under \(\mathscr {H}_1\) , the posterior distribution of \(\beta _j\) is
Suppose \(\beta _j^*\) is a maximum of f , we can calculate the negative Hessian at \(\beta _j^*\)
Then, the approximate posterior can be written as \(Q(\beta _j) = \mathcal {N}(\beta _j | \beta _j^*, A^{-1})\) . Thus, we can approximate the marginal likelihood
A larger value of \(\text {BF}_j\) suggests our preference for the hypothesis \(\mathscr {H}_1\) to the hypothesis \(\mathscr {H}_0\) , implying a potential key role of \({\textbf {x}}_j\) when predicting \({\textbf {z}}\) . Then after calculating \(\{\text {BF}_j, j=1,\cdots ,p\}\) , we can choose the top ranked variables with respect to \(\text {BF}_j\) . Say we select p explantory variables out of \(p^*\) variables. Next, we use these p selected variables \(\varvec{ x}\) for the Bayesian nonparametric classification model.
Stage II: BMARS-based classification modeling
In stage 2, we use a flexible nonlinear method to relate the response z with the selected explanatory variables from step 1. More specifically, we use Bayesian multivariate adaptive regression splines (BMARS) 27 , 28 which is a Bayesian version of a flexible non-parametric regression and classification method named MARS 40 . We extend the previously defined linear probit model for nonlinear modeling using product spline basis functions. We use the probit model defined in the previous section, for the i th observation \(p(z_{i}=1|y_{i})=\Phi (y_{i}), (i=1,\cdots ,n)\) . Next we use BMARS to relate the auxilary variables y with the explanatory variables \({\textbf {x}}\) through a regression model. In BMARS, for regression tasks, the product-based spline basis functions are not only used to model the unknown function f , but also automatically select the nonlinear interactions among the variables. The mapping function between the selected variables \({\textbf {x}}_i \in \mathscr {R}^p\) and the auxiliary variable \(y_i\) as below
where m is the number of basis functions and \(\alpha _j\) denotes the coefficient for the basic function \(B_j\) which is designed as
where the \(s_{qj} \in \{-1,1\}\) , the v ( q , j ) denotes the index of the variables and the set \(\{v(q,j);q=1,\cdots ,Q_j\}\) are not repeated, the \(t_{qj}\) refers to the partition location, \((\cdot )_+ = \max (0,\cdot )\) , and \(Q_j\) is the polynomial degree of the basic function \(B_j\) and also indicates the number of variables involved in \(B_j\) .
For probit model, the posterior distribution is not available in explicit form so we use Markov Chain Monte Carlo (MCMC) algorithm to simulate from the posterior distribution. As the dimension of the model m is unknown, we use the reversible jump Metropolis-Hastings algorithm 41 . More specifically, the model parameters we are interested in within the Bayesian framework of BMARS 27 are assumed to include the number of basis functions m , as well as their degree of interaction \(Q_j\) , their coefficients \(\alpha _j\) , their associated split points \(t_{qj}\) , and the sign indicators \(s_{qj}\) . We can use \(\varvec{\theta }^{(m)} = \{ \mathscr {B}_1,\cdots ,\mathscr {B}_m \}\) where \(\mathscr {B}_j\) to denote the model parameters \((Q_j, \alpha _j, t_{1j}, \cdots , t_{Q_j,j}, s_{1j}, \cdots , s_{Q_j,j})\) for each basis function \(B_j\) . Then, the hierarchical model can be written as
and the joint posterior for parameters m and \(\varvec{\theta }^{(m)}\) can be written in the following factorized form
In this algorithm, we update the model randomly using one of three steps, including (a) changing a node position, (b) creating a basis function, or (c) deleting a basis function, and then correcting the proposed new sample by the Metropolis-Hastings step 42 , 43 . Under this sampling scheme, samples based on significant variables are more likely to be accepted, which enables automatic feature selection by the algorithm and is important for us to make policy implications.
Data description
To understand vaccine impediments, we analyze a dataset obtained from one of the major health insurance providers in the United States. Since the dataset comes from the insured population, our analysis of impediments to vaccination and potential policy implications focuses on the insured population. More specifically, the dataset includes a total of 974,842 observations, each presenting information about one member of the insurance provider, with 1 binary response and 368 variables. About 69% of the variables are numeric and the remaining 31% are categorical. We note that we use synthetic data based on real data, which maintains all relationships within the dataset but is not specific to any individual insured person. This minimizes the risks associated with privacy to share protected data.
Response measures
The data records whether an insurance member is vaccinated or not. We assume that if a member is not vaccinated, then that member has some sort of impediment to vaccination. We use a broad definition of impediments that includes various reasons such as not believing in the efficacy of the vaccine, barriers like lack of resources, inability, or ideological/political reasons, etc.
The data document a number of characteristics of insured members that are potential variables influencing their willingness and availability to receive vaccines. These variables can be categorized into eight groups of characteristics, including medical claims, pharmacy claims, laboratory claims, demographics, credit data, condition-related data, centers for Medicare & Medicaid services (CMS) features (original reasons for entry into Medicare), and other characteristics. In total, there are 253 numerical variables and 115 categorical variables. The detailed descriptions of each group are provided in Table 1 .
Data pre-processing
Before modeling the data, we use some pre-processing steps to make the data structure compatible with the model. First, for convenience, we transform each categorical variable into several dummy variables. Thus, when the data are put into the model, there are 898 variables. Second, to fairly compare the different models, we balance the two types of samples by using a sample of 10,000 vaccinated clients and a sample of 10,000 unvaccinated clients as training data. Similarly, we sample a balanced test data including 2,000 clients.
Classification analysis: accuracy
In this section, in terms of accuracy, we compare our two-stage method (B-BMARS) with several widely-used classification models, including extreme gradient boosting (XGBoost) 44 , Gaussian process classification (GP) 45 , random forest (RF) 46 , and multilayer-perceptrons-based deep neural network (DNN) 47 , which have all demonstrated good performance in various applications. We use 0.5 as the threshold to calculate the accuracy, which is widely used for binary classification. For the overall analysis with different thresholds, we further use the area under curve (AUC) values 48 for comparison (shown in the next Section). Specifically for our B-BMARS, in the first stage, we use Bayes factor to quickly examine the potential predictive power of each variable on the response. Then, in the second stage, we use B-BMARS to fit the unknown function between the key variables and the responses in a more refined manner. A detailed description can be found in the “ Methods ” section.
In the first stage of pre-screening, we experiment by keeping different numbers of the top variables where the pre-screening dimension \(p_{scr} =50\) which is a proper value found empirically. We also compare the scenario without using pre-screening which corresponds to using all the 898 variables. However, using all the 898 variables is not practical with limited computational resources. Table 2 shows the accuracy among pre-screening dimension \(p_{scr}=50\) and without the pre-screening step. Our proposed B-BMARS gives the highest accuracy 0.614 and beats other popular baseline alternatives. Random Forest’s best result is close to our B-BMARS result but always below it.
We also visualize accuracy comparisons under pre-screening \(p_{scr}=50\) and scenario without pre-screening step in Fig. 2 a and b. The slash bars represent our B-BMARS, and the star bars represent XGBoost, GP, RF, and DNN from left to right, respectively. As we can see, the green bars are the highest in Fig. 2 a, and also comparable to the highest blue columns in Fig. 2 b. This indicates that our B-BMARS can maintain the performance for different scenarios. However, other baselines are relatively more sensitive to different settings. Additionally, we can see that RF achieves the best performance when \(p_{scr}=898\) , which leads to a high computational burden and is not practical with limited computational resources.
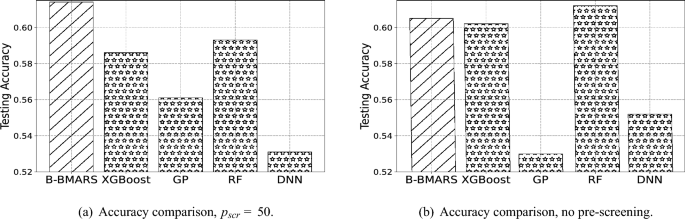
Visualization of accuracy comparison with baseline methods under pre-screening dimension \(p_{scr}=50\) and without pre-screening step. We compare our B-BMARS with extreme gradient boosting (XGBoost), Gaussian process classification (GP), random forest (RF), and multilayer-perceptrons-based deep neural network (DNN). Our B-BMARS generally improves or maintains accuracy compared to other baseline methods.
Classification analysis using AUC values
Apart from the accuracy, we also choose AUC values 49 to measure model performance, where higher AUC values indicate a better classifier. The baselines considered are aligned with the previous accuracy comparison. Table 3 shows the best AUC value among different pre-screening dimensions of each model. Our proposed B-BMARS gives the highest AUC value 0.651, followed by RF.
Similar to accuracy comparison, we also show detailed AUC value comparisons under pre-screening \(p_{scr}=50\) and scenario without pre-screening step in Fig. 3 a and b. The slash bars represent our B-BMARS, and the star bars represent XGBoost, GP, RF, and DNN from left to right, respectively. As shown in the figures, the green bars are the highest in Fig. 2 a, and also comparable to the highest blue columns in Fig. 2 b. This indicates that the classification rule from our B-BMARS is consistently one of the best classification rules in different pre-screening dimensions \(p_{scr}\) . However, other popular baselines have more fluctuations in different scenarios, with a drop in AUC values when resources are limited and pre-screening has to be used.
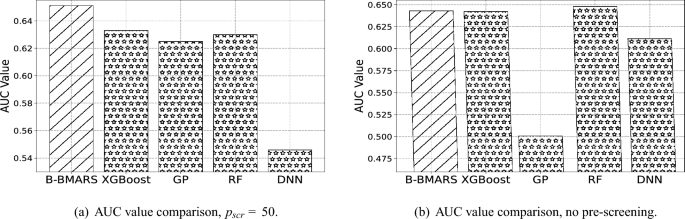
Visualization of the AUC value comparison with baseline methods under pre-screening dimension \(p_{scr}=50\) and without pre-screening step. We compare our B-BMARS with extreme gradient boosting (XGBoost), Gaussian process classification (GP), random forest (RF), and multilayer-perceptrons-based deep neural network (DNN). Our B-BMARS generally improves or maintains AUC value compared to other baseline methods.
Variable selection
B-BMARS is effective in selecting the most important variables. We find that there are four main categories of variables playing a key role in influencing the vaccine impediments of insured members, i.e., low household assets, high health risks, highly uninsured areas, and physician-related information. As shown in Table 4 , we list ten interesting and important variables selected by our B-BMARS, along with their detailed descriptions and the categories to which they belong.
When trying to determine people’s willingness or ability to take the COVID-19 vaccine, it is helpful to look at their household asset status, and we find that people with low household assets will be hesitant to receive the vaccine, which is in line with existing research findings 50 . For example, among the significant variables listed, Supplemental Nutrition Assistance Program (SNAP) benefits per capita is selected 20 , which reflects whether people generally have a stable source of food and thus reflects their household asset status. It is also important to check the number of non-mortgage accounts that are more than 60 days past due 51 . If many non-mortgage accounts are chronically past due, it is likely that household assets are low. In addition, we can see that per capita income in Table 4 in the last 12 months is one of the key variables, which gives a direct indication of people’s economic situation.
Health risk is another important variable of COVID-19 vaccination propensity prediction, and people are more reluctant to get vaccinated if they already have a high health risk 52 , 53 . For example, the trend in the number of prescriptions per month is noteworthy. It represents a change in people’s health status and can indicate whether they are at high health risk. In addition, we need to look at the number of monthly prescriptions related to heart disease-heart failure medications, which also shows how often people are taking their medications and revealing their health status.
In addition to the categories mentioned above, the availability of better healthcare coverage in the area also affects people’s proclivity to get vaccinated, and populations living in highly uninsured areas are more unlikely to receive COVID-19 vaccination 54 . As listed in the key variables, the net monthly payments for behavioral health claims related to skilled nursing inpatient facilities have a significant impact. Also, trends in monthly prescription costs associated with vaccine drugs reflect health care coverage and indicate people’s attitudes to vaccinations, and the percentage of adults under age 65 without health insurance in the corresponding area is selected. The higher the percentage, the worse the health care coverage is.
Last but not least, there is a need to consider whether individuals trust their physicians and the public health system 55 , 56 ; if individuals are not willing to use their physicians as their primary source of medical information, they are unlikely to be vaccinated 57 . For instance, we can get some information about people’s beliefs from the percentage of physician evaluations and claims management related to outpatient visits in the past year. A relatively high percentage score means that people are more likely to use their public health system and trust their doctors.
Non-linear basic function selection
To provide more interpretation of the selected variables, we plot a nonlinear basis function curve including the selected variables. We take two important variables, namely the trend of the number of prescriptions and per capita income, as examples. As depicted in Fig. 4 a, the propensity to vaccinate begins to decline with increasing prescriptions when a small number of prescriptions is reached, indicating high health risks. In Fig. 4 b, people first become more willing to vaccinate and then gradually become less willing to vaccinate as their per capita income increases, consistent with what we observed in our data overview.
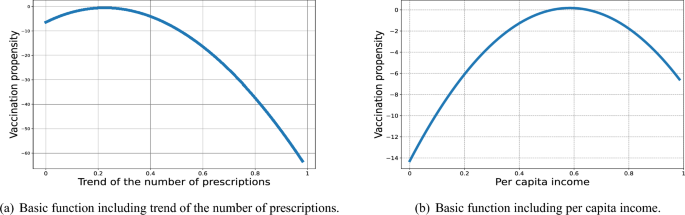
Curves of the non-linear basic functions selected by B-BMARS ( a ) trend of the number of prescriptions (Prescription Number Trend), and ( b ) per capita income (Per Capita Income).
Interaction selection
B-BMARS is not only effective in selecting the most important variables but also in identifying variables that have significant interactions. As shown in Table 5 , we list five important variable interactions selected by B-BMARS to predict COVID-19 vaccination propensity. Prescription count for cardiology is the most important and has many interactions with other variables 58 . Other variables are composed of health-related variables and financial conditions. Therefore, it is necessary to consider both the information related to the number of prescriptions and other important variables.
Business analysis and policy implication
Reducing vaccination impediments is important to slow the emergence of new virus variants. This will reduce the burden on patients and public health resources and will reduce costs incurred by insurance and healthcare providers. Thus, it is critical to develop targeted strategies to improve the ability to get vaccinated and reduce hesitancy. Vaccine impediment is a complex decision-making process influenced by a variety of contextual, individual and group, and vaccine-specific variables, including communication, socioeconomics, geographic barriers, vaccination experience, risk perception, and vaccination program design.
From our analysis in Section Variable Selection and Interaction Selection, it is clear that people with low assets, high health risks, low medical coverage, and distrust of doctors and the public health system are mostly reluctant to get vaccinated. Moreover, according to our analysis, these characteristics interact with each other. For example, people with low assets or low medical coverage have higher health risks. For these members, there are greater barriers than for other members. They may have fewer resources, more difficulty reaching vaccination sites, and less information about the nature of the pandemic.
We define the cause of COVID-19 vaccination impediments in these groups as due to physical barriers, psychological barriers, and health barriers. Physical barriers can be explained by having less access to the vaccine. Members with disabilities are likely to suffer from a lack of mobility. Psychological barriers can be explained by misunderstanding and mistrust. Members with few assets, low income, and high debt may live in communities where mistrust is prevalent or have fewer resources to obtain accurate information about the vaccine. Health barriers can be explained by high health risks, such as chronic diseases. The members may be older, living insecurely, or in poor health and worried about the side effects of vaccination.
Physical barrier
Potential policy implications to overcome challenges to access vaccination. People tend not to get vaccinated if it is difficult and cumbersome to obtain the vaccination. Difficulties often arise from limited mobility due to disability or age, availability of time, transportation, and low supply of vaccinations.
Implication 1: For people with limited mobility due to disability or age, we do not recommend that they visit a medical facility for vaccination, where there may be a high risk of cross-infection. We recommend that the health care provider provide home care services to help them get vaccinated at home.
Implication 2: For people with limited time, lack of transportation, and selected constraints, access to vaccines is limited for these and other reasons like poor financial status. The health care provider can provide them with travel assistance, such as language instruction and transportation help. The provider can also arrange some special activities including vaccination camps near their homes to help them get vaccinated.
Implication 3: For people in areas with low vaccine supply, it is more difficult for them to get vaccinated, even if they want to. Therefore, it is necessary to increase vaccine supply and reduce geographical inequalities. We recommend that the health care provider work with pharmacies to open more vaccination sites and productively send notifications to residents when vaccines are available.
Psychological barrier
Potential policy implications to overcome misunderstanding and mistrust of vaccine. People tend not to get vaccinated if they have misconceptions about the vaccine and think it will be harmful to them. The source of these misconceptions can be family, friends, social media, or social norms. Or they ignore the need for vaccination because they are currently in good health.
Implication 1: We recommend the health care provider get involved in community events and health activities to build stronger relationships with insured members. Then, it can select community leaders as vaccine ambassadors to deliver messages that allow vaccine recipients to share their reasons for vaccination, which will encourage people to reframe how they think about vaccines and build trust in the public health system.
Implication 2: For people who do not understand the necessity of vaccination, they may not be motivated enough to get vaccinated because they are in good health. We recommend emphasizing the age-independent health benefits and importance of vaccination, providing them instructional videos or organizing lectures.
Health barrier
Potential policy implications to overcome high health risk. People are more concerned about the side effects of vaccines if they are at high health risk. Specifically, they were concerned that the side effects of the vaccine would exacerbate their existing health problems.
Implication 1: We recommend obtaining more information about their health to understand if the vaccine can negatively interact with their current medications and existing problems. They should be educated if the vaccine is indeed safe for them.
Implication 2: We recommend using telemedicine to track their health after vaccination. This can prevent any unexpected health problems and make them feel more confident in the public health system.
Expected benefit analysis
In this section, we use an example to analyze the expected benefits of utilizing our methodology and the resulting policy implications to address vaccine impediments. We have actual data from a major U.S. healthcare provider. It is a publicly listed company that is committed to maximizing benefits for its stakeholders, particularly its shareholders. Given the data available to us, and utilizing publicly available data from other sources, we would like to estimate the preventable costs and incremental costs associated with our proposal to determine the impact on the savings of the healthcare provider.
More specifically, we use an all-vaccination rate (VRate) of 19.55% as of March 31st, 2021, which is derived by dividing the number of U.S. all-vaccinated persons by the U.S. population. The number of U.S. all-vaccinated persons is 64,852,669 from the Centers for Disease Control and Prevention’s (CDC) COVID data tracker 59 , and the U.S. population is 331,791,631 according to the United States Census Bureau’s U.S. and World Population Clock 60 . In addition, we use a number of Medicare Advantage members of one of the major health insurance providers in the United States (N) of 4,600,000 in 2020. Therefore, we can approximate the number of impedimented members as \(\text {N}_u\) = (1-VRate) \(\cdot\) N=3,700,700.
We then calculate the amount of preventable costs based on our B-BMARS method by encouraging more members to get vaccinated. From Centers for Medicare and Medicaid Services (CMS) report 61 , we obtain the average cost of medical services for COVID-19 hospitalizations and the number of medicare hospitalizations due to COVID-19 per 100,000 patients, which are $24,000 and 1,825, respectively. We also get the effectiveness of full vaccination in preventing hospitalization. According to the CDC’s August 2021 presentation in Morbidity and Mortality Weekly Report 62 , the effectiveness in adults 75 years or older is 91% for Pfizer-BioNTech, 96% for Moderna, and 85% for Janssen COVID-19 vaccines(CDC). Therefore, we choose 85% to approximate the lower bound of savings. Using our B-BMARS (as shown in Table 2 ), we are able to successfully identify 61.4% of impedimented members ( \(\text {R}_s\) ). Based on this information, we calculate the preventable costs for patients not vaccinated with COVID-19 via Equation ( 9 ). Specifically, the \(\text {Hospital}/100,000\) calculates the percentage of people who receive Medicare hospitalizations. We multiply this by \(\text {R}_s\) and \(\text {Ratio}\) to represent the approximate proportion of people spared hospitalization by vaccination. We then multiply this by \(\text {N}_u\) to get the approximate number of hospitalizations prevented by vaccination. Finally, we multiply this by \(\text {Fee}\) , which is the cost of patients preventable through vaccination. The results are shown in Table 6 , and we successfully prevent more than 845 million dollars in costs.
In addition, we approximate the extra cost of the incremental vaccination. We collect relevant information from Centers for Medicare and Medicaid Services (CMS) 63 . Specifically, for those without disabilities, the cost of the vaccination is $80 per person, assuming 2 doses of each vaccine and a single dose cost to Medicare of $40. For those with disabilities, the cost of the home vaccination has increased to $150 per person because of an additional $35 per dose. In our dataset, the percentage of people with disabilities is 25%. Therefore, we can estimate the cost of having impedimented members vaccinated following Equation ( 10 ). Specifically, we multiply the ratios \((1-\text {R}_d)\) and \(\text {R}_d\) by \(\text {N}_u\) to give the approximate numbers of people without and with disabilities, respectively. Next, we multiply these two numbers by \(\text {R}_s\) to get a rough estimate of the number of people in each impedimented group that we can successfully identify. This is then multiplied by the corresponding costs \(\text {Cost}_{nd}\) and \(\text {Cost}_{d}\) , respectively. Finally, we add the estimated costs of the two groups to arrive at the final total extra cost. The result is $221,542,406 as shown in Table 7 .
Based on all the above calculations, using Equation ( 11 ), we obtain a total savings of more than $ 624 million by addressing impediments to vaccination for the insured population. Specifically, we subtracted the total extra cost of vaccination from the total preventable costs due to vaccination to derive the total savings. We do not have a firm estimate of the marginal cost of implementing our policy recommendations. However, we are informed that it will be a small fraction of the $624 million savings calculated here. At a minimum, this number provides health insurance and healthcare providing organization guidance in developing a budget for implementing our policy recommendations. This example demonstrates how our methods can be transformed to a monetary value.
In this paper, we propose a flexible Bayesian method for predicting COVID-19 vaccination impediment scores under a Bayesian paradigm. Based on the accuracy of the results, we conclude that our proposed forecasting method performed better than the existing cutting-edge methods. The key findings of this study are:
The proposed method, B-BMARS performed better than XGBoost, Gaussian Process, and Random Forest in terms of classification accuracy.
Several important groups of variables are identified which could be the reasons for vaccine impediment, e.g., health risk and healthcare coverage.
We identified four main categories of variables playing a key role in influencing the attitude of the public towards vaccines, including low household assets, high health risks, highly uninsured areas, and infrequent use of physician information.
Interactions among some of these variables may play a crucial role in vaccine impediment, e.g. combining low medical coverage and low assets have more prediction power for vaccination impediment.
We define the cause of COVID-19 vaccination impediments in these groups as due to physical barriers, psychological barriers, and health barriers. We then provide policy recommendations to reduce barriers from the perspective of each of these three barriers.
Physical barriers can be explained by having less access to the vaccine, e.g., limited mobility, limited time, lack of transportation, and low vaccine supply. To overcome such barriers, we recommend that health care providers offer home care services, travel assistance, and arrange for special events, including vaccination camps.
Psychological barriers refer to misconceptions or neglect of vaccines and can come from family, friends, social media, social norms, or good health. To overcome these barriers, we recommend that health care providers engage in community events and wellness activities, build stronger relationships and trust with insured members, and provide them with instructional videos or organize lectures that emphasize the health benefits and importance of vaccination.
Health barriers are people’s existing health problems that make them more worried about the side effects of vaccines. To overcome such barriers, we recommend that health care providers obtain more information about people’s health, provide more specific advice to each individual, and use telemedicine to track their health after vaccination.
We estimated the dollar benefit based on actual data and publicly available information resulting from our potential policy implications.
To the best of our knowledge, this is the first research that uses these flexible methods to analyze the data and arrive at conclusions that will have a significant impact on corporate decision making. Our findings have broad implications for solving complex problems with large datasets that require forecasting. Finally, our framework can have a direct impact on corporate and public policy related to future pandemics.
For future research, it is of great importance to expand the study to uninsured members and barriers in other countries. Equally important is how other types of data (e.g., image and text data), if available, can be incorporated to further improve predictive accuracy, better address vaccine barriers, and provide additional benefits. Additionally, we plan to enhance the scalability of the algorithm by employing parallel Markov Chain Monte Carlo (MCMC) within a simulated annealing framework 64 . This approach aims to enable the implementation of the algorithm in a single stage.
Data availability
The data files of the customer’s vaccine intentions and characteristics are available upon reasonable request from Prof. Mahajan ([email protected]).
The center for systems science and engineering (csse) at johns hopkins university: Covid-19 content portal. www.systems.jhu.edu/research/public-health/ncov/ .
Haug, N. et al. Ranking the effectiveness of worldwide covid-19 government interventions. Nat. Hum. Behav. 4 , 1303–1312 (2020).
Article PubMed Google Scholar
Mavragani, A. & Gkillas, K. Exploring the role of non-pharmaceutical interventions (npis) in flattening the greek covid-19 epidemic curve. Sci. Rep. 11 , 11741 (2021).
Article ADS CAS PubMed PubMed Central Google Scholar
Giordano, G. et al. Modelling the covid-19 epidemic and implementation of population-wide interventions in Italy. Nat. Med. 26 , 855–860 (2020).
Article CAS PubMed PubMed Central Google Scholar
Kwame, A., Makarova, V., Hudu, F. & Petrucka, P. M. The covid-19 pandemic in ghana: Exploring the discourse strategies in president nana addo’s speeches. Humanit. Soc. Sci. Commun. 10 , 1–10 (2023).
Article Google Scholar
Allen, D. W. Covid-19 lockdown cost/benefits: A critical assessment of the literature. Int. J. Econ. Bus. 29 , 1–32 (2022).
Matzinger, P. & Skinner, J. Strong impact of closing schools, closing bars and wearing masks during the covid-19 pandemic: Results from a simple and revealing analysis. medRxiv (2020).
Saqib, M. A. N. et al. Effect of covid-19 lockdown on patients with chronic diseases. Diabetes Metab. Syndr. Clin. Res. Rev. 14 , 1621–1623 (2020).
Orgilés, M., Morales, A., Delvecchio, E., Mazzeschi, C. & Espada, J. P. Immediate psychological effects of the covid-19 quarantine in youth from Italy and Spain. Front. Psychol. 11 , 2986 (2020).
Krekel, C., Swanke, S., De Neve, J.-E. & Fancourt, D. Happiness predicts compliance with preventive health behaviours during Covid-19 lockdowns. Sci. Rep. 13 , 7989 (2023).
Principi, N. & Esposito, S. Why it is important to develop an effective and safe pediatric covid-19 vaccine. Vaccines 9 , 127 (2021).
Sallam, M. Covid-19 vaccine hesitancy worldwide: A concise systematic review of vaccine acceptance rates. Vaccines 9 , 160 (2021).
Biswas, N., Mustapha, T., Khubchandani, J. & Price, J. H. The nature and extent of covid-19 vaccination hesitancy in healthcare workers. J. Community Health 46 , 1244–1251 (2021).
Article PubMed PubMed Central Google Scholar
Machingaidze, S. & Wiysonge, C. S. Understanding covid-19 vaccine hesitancy. Nat. Med. 27 , 1338–1339 (2021).
Article CAS PubMed Google Scholar
Solís Arce, J. S. et al. Covid-19 vaccine acceptance and hesitancy in low-and middle-income countries. Nat. Med. 27 , 1385–1394 (2021).
Dubé, E. & MacDonald, N. E. Covid-19 vaccine hesitancy. Nat. Rev. Nephrol. 18 , 409–410 (2022).
Lazarus, J. V. et al. Revisiting covid-19 vaccine hesitancy around the world using data from 23 countries in 2021. Nat. Commun. 13 , 3801 (2022).
Macharia, J. M. et al. An empirical assessment of the factors influencing acceptance of covid-19 vaccine uptake between Kenyan and Hungarian residing populations: A cross-sectional study. Sci. Rep. 12 , 22262 (2022).
Article ADS PubMed PubMed Central Google Scholar
Lazarus, J. V. et al. A survey of covid-19 vaccine acceptance across 23 countries in 2022. Nat. Med. 29 , 366–375 (2023).
Acharya, B. & Dhakal, C. Implementation of state vaccine incentive lottery programs and uptake of covid-19 vaccinations in the united states. JAMA Netw. Open 4 , e2138238–e2138238 (2021).
Nguyen, L. H. et al . Racial and ethnic differences in covid-19 vaccine hesitancy and uptake. medrxiv (2021).
Nguyen, K. H. et al. Disparities in national and state estimates of covid-19 vaccination receipt and intent to vaccinate by race/ethnicity, income, and age group among adults ≥ 18 years, United States. Vaccine 40 , 107–113 (2022).
Kini, A. et al. Differences and disparities in seasonal influenza vaccine, acceptance, adverse reactions, and coverage by age, sex, gender, and race. Vaccine 40 , 1643–1654 (2022).
Sypsa, V. et al. Trends in covid-19 vaccination intent, determinants and reasons for vaccine hesitancy: Results from repeated cross-sectional surveys in the adult general population of Greece during November 2020-June 2021. Vaccines 10 , 470 (2022).
Hoijtink, H., Mulder, J., van Lissa, C. & Gu, X. A tutorial on testing hypotheses using the Bayes factor. Psychol. Methods 24 , 539 (2019).
Keysers, C., Gazzola, V. & Wagenmakers, E.-J. Using Bayes factor hypothesis testing in neuroscience to establish evidence of absence. Nat. Neurosci. 23 , 788–799 (2020).
Denison, D. G., Mallick, B. K. & Smith, A. F. Bayesian mars. Stat. Comput. 8 , 337–346 (1998).
Lei, B. et al. Bayesian optimization with adaptive surrogate models for automated experimental design. Npj Comput. Mater. 7 , 194 (2021).
Article ADS Google Scholar
Holmes, C. C., Denison, D. G. T. & Mallick, B. K. Accounting for model uncertainty in seemingly unrelated regressions. J. Comput. Graph. Stat. 11 , 533–551, 3 (2002).
McCullagh, P. Generalized Linear Models (Routledge, 2019).
Albert, J. H. & Chib, S. Bayesian analysis of binary and polychotomous response data. J. Am. Stat. Assoc. 88 , 669–679 (1993).
Article MathSciNet Google Scholar
Hong, S., Kim, Y. & Park, T. Practical issues in screening and variable selection in genome-wide association analysis. Cancer Inform. 13 , CIN–S16350 (2014).
Kirpich, A. et al. Variable selection in omics data: A practical evaluation of small sample sizes. PloS One 13 , e0197910 (2018).
Ghosh, A. & Thoresen, M. A robust variable screening procedure for ultra-high dimensional data. Stat. Methods Med. Res. 30 , 1816–1832 (2021).
Article MathSciNet PubMed Google Scholar
Schönbrodt, F. D. & Wagenmakers, E.-J. Bayes factor design analysis: Planning for compelling evidence. Psychon. Bull. Rev. 25 , 128–142 (2018).
Liang, F., Paulo, R., Molina, G., Clyde, M. A. & Berger, J. O. Mixtures of g priors for Bayesian variable selection. J. Am. Stat. Assoc. 103 , 410–423 (2008).
Article MathSciNet CAS Google Scholar
Daxberger, E. et al. Laplace redux-effortless Bayesian deep learning. Adv. Neural Inf. Process. Syst. 34 , 20089–20103 (2021).
Google Scholar
Girolami, M. & Rogers, S. Variational Bayesian multinomial probit regression with gaussian process priors. Neural Comput. 18 , 1790–1817 (2006).
Kuss, M., Rasmussen, C. E. & Herbrich, R. Assessing approximate inference for binary Gaussian process classification. J. Mach. Learn. Res. 6 (2005).
Friedman, J. H. Multivariate adaptive regression splines. Ann. Stat. 1–67 (1991).
Green, P. J. Reversible jump Markov chain monte Carlo computation and Bayesian model determination. Biometrika 82 , 711–732 (1995).
Denison, D. G., Holmes, C. C., Mallick, B. K. & Smith, A. F. Bayesian methods for nonlinear classification and regression, vol. 386 (John Wiley & Sons, 2002).
Holmes, C. & Mallick, B. Generalized nonlinear modeling with multivariate free-knot regression splines. J. Am. Stat. Assoc. 98 , 352–368 (2003).
Chen, T. et al . Xgboost: Extreme gradient boosting. R package version 0.4-2 1 , 1–4 (2015).
Nickisch, H. & Rasmussen, C. E. Approximations for binary Gaussian process classification. J. Mach. Learn. Res. 9 , 2035–2078 (2008).
MathSciNet Google Scholar
Breiman, L. Random forests. Mach. Learn. 45 , 5–32 (2001).
Almeida, L. B. Multilayer perceptrons. In Handbook of Neural Computation , C1–2 (CRC Press, 2020).
Huang, J. & Ling, C. X. Using auc and accuracy in evaluating learning algorithms. IEEE Trans. Knowl. Data Eng. 17 , 299–310 (2005).
Article CAS Google Scholar
Ling, C. X., Huang, J. & Zhang, H. Auc: a better measure than accuracy in comparing learning algorithms. In Conference of the Canadian Society for Computational Studies of Intelligence , 329–341 (Springer, 2003).
Kadoya, Y. et al. Willing or hesitant? A socioeconomic study on the potential acceptance of covid-19 vaccine in Japan. Int. J. Environ. Res. Public Health 18 , 4864 (2021).
Biddle, N., Edwards, B., Gray, M. & Sollis, K. Change in vaccine willingness in Australia: August 2020 to January 2021. MedRxiv 2021–02 (2021).
Warren, A. M., Perrin, P. B., Elliott, T. R. & Powers, M. B. Reasons for covid-19 vaccine hesitancy in individuals with chronic health conditions. Health Sci. Rep. 5 (2022).
Ku, L. The association of social factors and health insurance coverage with covid-19 vaccinations and hesitancy, July 2021. J. Gen. Intern. Med. 37 , 409–414 (2022).
Allen, J. D., Abuelezam, N. N., Rose, R. & Fontenot, H. B. Factors associated with the intention to obtain a covid-19 vaccine among a racially/ethnically diverse sample of women in the usa. Transl. Behav. Med. 11 , 785–792 (2021).
Paul, E., Steptoe, A. & Fancourt, D. Attitudes towards vaccines and intention to vaccinate against covid-19: Implications for public health communications. The Lancet Regional Health–Europe 1 (2021).
Dhalaria, P., Arora, H., Singh, A. K. & Mathur, M. Covid-19 vaccine hesitancy and vaccination coverage in India: An exploratory analysis. Vaccines 10 , 739 (2022).
Holroyd, T. A. et al. Development of a scale to measure trust in public health authorities: Prevalence of trust and association with vaccination. J. Health Commun. 26 , 272–280 (2021).
Bogart, L. M. et al . Covid-19 related medical mistrust, health impacts, and potential vaccine hesitancy among black Americans living with HIV. J. Acquir. Immune Defic. Syndr. (1999) 86 , 200 (2021).
Centers for disease control and prevention report: Trends in number of covid-19 vaccinations in the us. www.covid.cdc.gov/covid-data-tracker/#vaccination-trends .
United states census bureau: U.S. and world population clock. www.census.gov/popclock/ .
Centers for medicare and medicaid services: Medicare covid-19 data snapshot. www.cms.gov/files/document/medicare-covid-19-data-snapshot-services-through-2021-03-20.pdf .
Centers for disease control and prevention report: Morbidity and mortality weekly report. www.cdc.gov/mmwr/volumes/70/wr/mm7032e3.htm?s_cid=mm7032e3_w .
Centers for medicare and medicaid services: Medicare covid-19 vaccine shot payment. www.cms.gov/medicare/payment/covid-19/medicare-covid-19-vaccine-shot-payment .
Payne, R. D., Guha, N. & Mallick, B. K. A Bayesian survival tree partition model using latent Gaussian processes. Biometrics, To appear (2024).
Download references
Acknowledgements
We thank Vinay Chiguluri, Sravya Etlapur, Andrew J. Fieldhouse, and Geoffrey Monsees for valuable comments, and Xiaan Zhou for capable research assistance.
Author information
Authors and affiliations.
Department of Statistics, Texas A&M University, College Station, TX, USA
Bowen Lei & Bani Mallick
Department of Finance, Texas A&M University, College Station, TX, USA
Arvind Mahajan
You can also search for this author in PubMed Google Scholar
Contributions
B.L. and B.M. conceived the concept of B-BMARS. B.L. implemented the algorithm and performed the experiments. AM provided data files on the vaccine intentions of the customers and provided business and financial background. All authors analyzed the results, contributed to the manuscript, and edited it. All authors reviewed the final version of the manuscript.
Corresponding author
Correspondence to Bani Mallick .
Ethics declarations
Competing interests.
The authors declare no competing interests.
Additional information
Publisher's note.
Springer Nature remains neutral with regard to jurisdictional claims in published maps and institutional affiliations.
Supplementary Information
Supplementary information., rights and permissions.
Open Access This article is licensed under a Creative Commons Attribution 4.0 International License, which permits use, sharing, adaptation, distribution and reproduction in any medium or format, as long as you give appropriate credit to the original author(s) and the source, provide a link to the Creative Commons licence, and indicate if changes were made. The images or other third party material in this article are included in the article’s Creative Commons licence, unless indicated otherwise in a credit line to the material. If material is not included in the article’s Creative Commons licence and your intended use is not permitted by statutory regulation or exceeds the permitted use, you will need to obtain permission directly from the copyright holder. To view a copy of this licence, visit http://creativecommons.org/licenses/by/4.0/ .
Reprints and permissions
About this article
Cite this article.
Lei, B., Mahajan, A. & Mallick, B. Identifying and overcoming COVID-19 vaccination impediments using Bayesian data mining techniques. Sci Rep 14 , 8595 (2024). https://doi.org/10.1038/s41598-024-58902-1
Download citation
Received : 23 November 2023
Accepted : 04 April 2024
Published : 13 April 2024
DOI : https://doi.org/10.1038/s41598-024-58902-1
Share this article
Anyone you share the following link with will be able to read this content:
Sorry, a shareable link is not currently available for this article.
Provided by the Springer Nature SharedIt content-sharing initiative
By submitting a comment you agree to abide by our Terms and Community Guidelines . If you find something abusive or that does not comply with our terms or guidelines please flag it as inappropriate.
Quick links
- Explore articles by subject
- Guide to authors
- Editorial policies
Sign up for the Nature Briefing newsletter — what matters in science, free to your inbox daily.

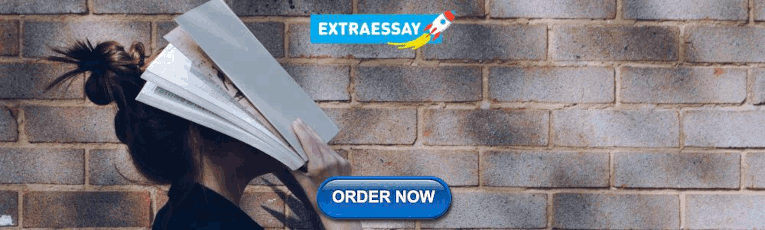
IMAGES
COMMENTS
The World Health Organization (WHO) declared the COVID-19, a pandemic in March 2020 [ 1 ]. The SARS-CoV-2 has affected over 105 million people and has claimed over 2.29 million lives worldwide, as of February 5, 2021. The most affected countries have been the United States of America, with over 26.7 million cases and 456,000 deaths, and India ...
In Fig. 2, the overview of the global COVID-19 vaccine landscape in clinical development depicts that there are seven major types of vaccine candidates for COVID-19 is illustrated as (inactivated, non-replicating viral vectors, replicating viral vectors, protein subunit, nucleic acid-based, and virus-like particles [VLP]), showing the percentage of candidate vaccines that are currently under ...
The development of COVID-19 vaccines is mainly based on seven platforms, which can be classified into three modes according to the antigen category. 17,18 The first mode is based on the protein ...
In addition, the development of other prominent COVID-19 vaccines will be highlighted alongside the sustainability of the vaccine-mediated immune response and current contraindications. As the research is rapidly expanding, we have looked at the association between pregnancy and COVID-19 vaccinations, in addition to the current reviews on the ...
With this in mind, the editors of NPJ Vaccines have selected 17 articles that exemplify the rapid progress made with COVID vaccine development in the last 2 years. Our first COVID paper described ...
VOL. 387 NO. 11. The coronavirus disease 2019 (Covid-19) pandemic has claimed an estimated 15 million lives, including more than 1 million lives in the United States alone. The rapid development ...
COVID-19 vaccine R&D landscape. As of 8 April 2020, the global COVID-19 vaccine R&D landscape includes 115 vaccine candidates (Fig. 1), of which 78 are confirmed as active and 37 are unconfirmed ...
Discussion. A two-dose regimen of BNT162b2 (30 μg per dose, given 21 days apart) was found to be safe and 95% effective against Covid-19. The vaccine met both primary efficacy end points, with ...
Severe Acute Respiratory Syndrome Coronavirus 2 (SARS-CoV-2) is a new type of coronavirus that causes the Coronavirus Disease 2019 (COVID-19), which has been the most challenging pandemic in this century. Considering its high mortality and rapid spread, an effective vaccine is urgently needed to control this pandemic. As a result, the academia, industry, and government sectors are working ...
Parental intention on getting children COVID-19 vaccinations: Invariance evaluation across parenting roles and COVID-19-like symptoms experiences among Iranians during the pandemic period, Human ...
In this article we review the standard FDA approach to vaccine evaluation, which underpins its current approaches to assessment of vaccines to prevent coronavirus disease 2019 (COVID-19). The FDA has established pathways to accelerate vaccine availability before approval, such as Emergency Use Authorization, and to channel resources to high ...
The development of COVID-19 vaccines is mainly based on seven platforms, which can be classified into three modes according to the antigen category. 17,18 The first mode is based on the protein produced in vitro, including inactivated vaccines (inactivated SARS-CoV-2), VLP vaccines (virus particles without nucleic acid), and subunit vaccines (S ...
Years before the COVID-19 pandemic began, experts at the NIH Vaccine Research Center (VRC) were studying coronaviruses to find out how to protect against them. The scientists chose to focus on one "prototype" coronavirus and create a vaccine for it. That vaccine could then be customized to fight different coronaviruses.
In a human challenge vaccine study, healthy volunteers are given an experimental vaccine, and then deliberately exposed to the organism causing the disease to see if the vaccine works. Some scientists believe that this approach could accelerate COVID-19 vaccine development, in part because it would require far fewer volunteers than a typical study.
An mRNA vaccine candidate (Fig. 3l), termed mRNA-1273, is under development by the Vaccine Research Center ... This paper reports a first-in ... E. E. et al. RNA-based COVID-19 vaccine BNT162b2 ...
A booster of the COVID-19 vaccine targeting the prevailing Omicron variant did not become available in the United States until a year after the variant was first detected. This pattern of developing, testing, and distributing a variant-specific booster may become the default response to further waves of COVID-19 caused by new variants.
2020 has been a difficult year for all, but has seen 58 vaccines against severe acute respiratory syndrome coronavirus 2 (SARS-CoV-2) be developed and in clinical trials,1 with some vaccines reportedly having more than 90% efficacy against COVID-19 in clinical trials. This remarkable achievement is much-needed good news as COVID-19 cases are currently at their highest daily levels globally.2 ...
The Coronavirus Efficacy (COVE) phase 3 trial was launched in late July 2020 to assess the safety and efficacy of the mRNA-1273 vaccine in preventing SARS-CoV-2 infection. An independent data and ...
On August 23, 2021, the Pfizer-BioNTech was the first COVID-19 vaccine officially approved for commercialization by the FDA, 105 being also the first-ever approved on October 29, 2021 for use in children aged 5-11. 106 Thus, the mRNA vaccine technology has the most promising application prospects for COVID-19.
The final 40 papers included in this review consisted of 18 in 2021, 14 in 2022, and eight in 2023 ... Future studies on the economic evaluation of COVID-19 vaccines should prioritize virus typing and immune escape, ensure the timely update of research data, improve parameter quality, and incorporate real-world data to improve model accuracy ...
Keywords: COVID-19, SARS-CoV-2, vaccines, CoronaVac, review Citation: Alzate-Ángel JC, Avilés-Vergara PA, Arango-Londoño D, Concha-Eastman A, Garcés-Hurtado A, López-Carvajal L, Minotta IL, Ortega-Lenis D, Quintero G, Reina-Bolaños S, Reina-Bolaños CA, Roa P, Sánchez-Orozco M, Tovar-Acero C and Arbeláez-Montoya MP (2024) How has research on the effectiveness and safety of COVID-19 ...
With more than 60 vaccine candidates in clinical trials and another 170 in preclinical development (WHO COVID-19 vaccine ... policy for research and development of vaccines for public health ...
The rapid development of vaccines is a crucial objective in modern biotechnology and molecular pharmacology. In this context, conducting research to expedite the selection of a potent immunogen is imperative. The candidate vaccine should induce the production of antibodies that can recognize the immunogenic epitopes of the target protein, resembling the ones found in recovered patients.
2. Vaccination strategies. Many efforts have been directed towards the development of the vaccines against COVID-19, to avert the pandemic and most of the developing vaccine candidates have been using the S-protein of SARS-CoV-2 (Dhama et al., 2020).As of July 2, 2020, the worldwide SARS-CoV-2 vaccine landscape includes 158 vaccine candidates, out of which 135 are in the preclinical or the ...
WASHINGTON — A new report from the National Academies of Sciences, Engineering, and Medicine reviews evidence for 19 potential harms of the COVID-19 vaccines, and for nine potential shoulder injuries from intramuscular administration of vaccines more broadly. The committee that conducted the review identified sufficient evidence to draw 20 conclusions about whether these vaccines could cause ...
This study investigated the patterns of public discourse and message-level drivers of user reactions on X regarding HIV vaccines by analyzing posts using machine learning algorithms and highlighted COVID-19 as a significant context for public discourse and reactions regarding HIV vaccines from both positive and negative perspectives. BACKGROUND The initiation of clinical trials for messenger ...
In a major development, on January 3, 2021, DCGI approved two COVID-19 vaccines for restricted emergency use in the country 20. Bharat Biotech's Covid-19 vaccine Covaxin has been recommended for ...
The achievements of vaccine research and development bring a hope to our societies that we may cope with the COVID‐19 pandemic. There are two aspects that should be maintained in balance: the immediate necessity for speed of vaccine research and the inherent need for protection of research subjects, which is the foremost concern of research ethics.
The COVID-19 pandemic has profoundly reshaped human life. The development of COVID-19 vaccines has offered a semblance of normalcy. However, obstacles to vaccination have led to substantial loss ...