- Second Opinion
Fetal Growth Restriction
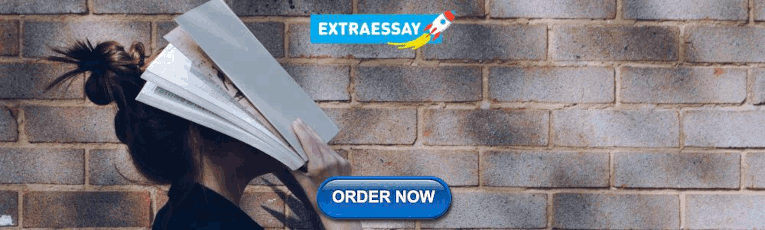
What is fetal growth restriction (FGR)?
Fetal growth restriction (FGR) is a condition in which an unborn baby (fetus) is smaller than expected for the number of weeks of pregnancy (gestational age). It is often described as an estimated weight less than the 10th percentile. This means that the baby weighs less than 9 out of 10 babies of the same gestational age. Newborn babies with FGR may be called “small for gestational age.”
FGR can begin at any time during pregnancy. With FGR, the baby does not grow well. FGR may affect the overall size of the baby and the growth of organs, tissues, and cells. This can cause many problems. But many newborns who are small may just be small. They may not have any problems.
What causes FGR?
Many things increase the risk for FGR. These include problems with the placenta or umbilical cord. The placenta may not attach well. Or the blood flow through the umbilical cord may be limited. Factors in both the mother and the baby may cause FGR.
Factors in the mother that can cause FGR include:
High blood pressure or other heart and blood vessel disease
Too few red blood cells (anemia)
Long-term lung or kidney conditions
Autoimmune conditions such as lupus
Very low weight
A large amount of excess weight (obese)
Poor nutrition or weight gain
Alcohol or drug use
Cigarette smoking
Factors in the baby that can cause FGR include:
Being one of a twin or triplets
Birth defects, such as heart defects
Problem with genes or chromosomes
What are the symptoms of FGR?
A pregnant woman doesn’t have symptoms of FGR. But a baby with FGR may have certain signs after birth, such as:
Low birth weight
Low blood sugar levels
Lower body temperature
High level of red blood cells
Trouble fighting infections
How is FGR diagnosed?
One of the main reasons for regular prenatal exams is to make sure your baby is growing well. During pregnancy, the size of your baby is estimated in different ways, including:
Fundal height. To check fundal height, your healthcare provider measures from the top of your pubic bone to the top of your uterus (fundus). Fundal height, measured in centimeters (cm), is about the same as the number of weeks of pregnancy after the 20th week. For example, at 24 weeks gestation, your fundal height should be close to 24 cm. If the fundal height is less than expected, it may mean FGR.
If your healthcare provider thinks you have FGR, you will have other tests. These include:
Fetal ultrasound. Estimating fetal weight with ultrasound is the best way to find FGR. Ultrasound uses sound waves to create images of the baby in the womb. Sound waves will not harm you or the baby. Your healthcare provider or a technician will use the images to measure the baby. A diagnosis of FGR is based on the difference between actual and expected measurements at a certain gestational age.
Doppler ultrasound. You may also have this special type of ultrasound to diagnose FGR. Doppler ultrasound checks the blood flow to the placenta and through the umbilical cord to the baby. Decreased blood flow may mean your baby has FGR.
You may have repeat ultrasound exams, Doppler studies, and other tests.
How is FGR managed?
Management depends on how serious the FGR is. This is based on the ultrasound (estimated fetal weight) and Doppler ultrasound (blood flow to the baby), as well is risk factors and the number of weeks gestation.
Treatment may include:
Frequent monitoring. This means you will have prenatal visits more often, and ultrasound and Doppler ultrasound exams. You may have other tests.
Tracking fetal movements. Your healthcare provider may also ask you to keep track of fetal movements. If so, he or she will give you instructions.
Corticosteroid medicine
Hospital stay
Early delivery or emergency cesarean
What are possible complications of FGR?
FGR can cause many serious complications. Your baby may need to be delivered early and stay in the hospital. Your baby may have trouble breathing, infections, and other problems. Stillbirths and death may occur. As your child grows, he or she will be at higher risk for heart and blood vessel problems.
How can FGR be prevented?
FGR can happen in any pregnancy. But some factors, like cigarette smoking or alcohol or medicine use, increase the risk for FGR. Regular and early prenatal care and a healthy diet and steady weight gain help to prevent FGR and other problems.
When should I call my healthcare provider?
Make sure your healthcare provider knows your health history. If you are counting fetal movements and find that the number has decreased, let your healthcare provider know. And if you notice other changes or if you have concerns about your pregnancy, call your healthcare provider.
Key points about fetal growth restriction
FGR is a condition in which the baby is smaller than expected for gestational age.
Many factors increase the risk for FGR. They may be related to the placenta, mother, or baby.
Estimating fetal weight with ultrasound is the best way to identify FGR.
If FGR is diagnosed, you will need to be closely monitored.
Tips to help you get the most from a visit to your healthcare provider:
Know the reason for your visit and what you want to happen.
Before your visit, write down questions you want answered.
Bring someone with you to help you ask questions and remember what your provider tells you.
At the visit, write down the name of a new diagnosis, and any new medicines, treatments, or tests. Also write down any new instructions your provider gives you.
Know why a new medicine or treatment is prescribed, and how it will help you. Also know what the side effects are.
Ask if your condition can be treated in other ways.
Know why a test or procedure is recommended and what the results could mean.
Know what to expect if you do not take the medicine or have the test or procedure.
If you have a follow-up appointment, write down the date, time, and purpose for that visit.
Know how you can contact your provider if you have questions.
Related Links
- Johnson Center
- Fetal and Pregnancy Health
- Pediatric Cardiology
- Our Services
- Chiari Malformation Center at Stanford Medicine Children's Health
Related Topics
Care of the Baby in the Delivery Room
Connect with us:
Download our App:
- Leadership Team
- Vision, Mission & Values
- The Stanford Advantage
- Government and Community Relations
- Get Involved
- Volunteer Services
- Auxiliaries & Affiliates
© 123 Stanford Medicine Children’s Health
Learn how UpToDate can help you.
Select the option that best describes you
- Medical Professional
- Resident, Fellow, or Student
- Hospital or Institution
- Group Practice
- Patient or Caregiver
- Find in topic
RELATED TOPICS
INTRODUCTION
This topic will discuss pregnancy management and outcome of FGR in singleton pregnancies. Screening and diagnosis of FGR and evaluation to determine the probable cause are reviewed separately. (See "Fetal growth restriction: Screening and diagnosis" and "Fetal growth restriction: Evaluation" .)
FGR in twin pregnancies is also reviewed separately. (See "Twin pregnancy: Routine prenatal care", section on 'Screening for fetal growth restriction and discordance' and "Selective fetal growth restriction in monochorionic twin pregnancies" .)
PRENATAL CARE
Goal — The focus of prenatal care in pregnancies with FGR is to identify those fetuses who are at highest risk of perinatal demise and thus may benefit from early delivery. Distinguishing the constitutionally small fetus from the fetus with pathologic growth can help avoid unnecessary interventions for the former and lead to appropriate monitoring and delivery timing of the latter. Unfortunately, this distinction is not always possible.
Fetal surveillance — The key parameters for fetal surveillance are:
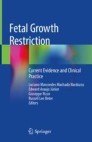
- © 2019
Fetal Growth Restriction
Current Evidence and Clinical Practice
- Luciano Marcondes Machado Nardozza 0 ,
- Edward Araujo Júnior 1 ,
- Giuseppe Rizzo 2 ,
- Russell Lee Deter 3
Paulista School of Medicine, Federal University of São Paulo, São Paulo, Brazil
You can also search for this editor in PubMed Google Scholar
University of Rome Tor Vergata, Roma, Italy
Baylor college of medicine, houston, usa.
- First book fully dedicated to Fetal Growth Restriction
- Encompasses state of the art research findings as well as new concepts and classifications
- Focuses on promoting a better prenatal assistance, avoiding obstetric complications and unwanted outcomes related to fetal growth restriction
10k Accesses
20 Citations
- Table of contents
About this book
Editors and affiliations, about the editors, bibliographic information.
- Publish with us
Buying options
- Available as EPUB and PDF
- Read on any device
- Instant download
- Own it forever
- Durable hardcover edition
- Dispatched in 3 to 5 business days
- Free shipping worldwide - see info
Tax calculation will be finalised at checkout
Other ways to access
This is a preview of subscription content, log in via an institution to check for access.
Table of contents (15 chapters)
Front matter, standards for fetal growth and neonatal growth outcomes.
- Russell L. Deter
Small for Gestational Age Versus Fetal Growth Restriction
Etiopathogeny.
- Anna Iacoi, Roland Axt-Fliedner
Physiopathology
- Gabriele Tonni, Edward Araujo Júnior, Maria Paola Bonasoni
Classification
- Talita Micheletti Helfer, Elisenda Eixarch, Francesc Figueras, Eduard Gratacós
- Ana Cristina Perez Zamarian, Jader de Jesus Cruz, Luciano Marcondes Machado Nardozza
Biochemical Assessment of Placental Function
- Irene Martín-Estal, Miguel Angel Rodriguez-Zambrano, Inma Castilla-Cortázar
Clinical Diagnosis
- Alberto Borges Peixoto, Laudelino Marques Lopes, Edward Araujo Júnior
Ultrasonography Diagnosis
- Nicola Fratelli, Cristina Zanardini, Federico Prefumo
Doppler Diagnosis
- Andrea Dall’Asta, Tullio Ghi, Tiziana Frusca
Clinical Treatment
- Luciano Marcondes Machado Nardozza, Ana Carolina Rabachini Caetano, Ana Cristina Perez Zamarian
Obstetric Management
- Ana Carolina Rabachini Caetano, Luciano Marcondes Machado Nardozza
Postnatal Prognosis
- Erich Cosmi, Matteo Andolfatto, Matteo Arata, Marilia Calanducci, Silvia Visentin
Neurological Complications
- Danilo Buca, Marco Liberati, Francesco D’Antonio
Maternal Cardiovascular Involvement
- Maya Reddy, Annie Kroushev, Kirsten Palmer, Daniel Rolnik, Fabricio Da Silva Costa
Back Matter
- Placental insufficiency
- Doppler management
- Swall for gestational age
- Maternal heart involvement
- Intrauterine growth restriction
- Intrauterine growth
- Fetal growth
- Neonatology
- First trimester
- Second trimester
- Third trimester
Luciano Marcondes Machado Nardozza, Edward Araujo Júnior
Giuseppe Rizzo
Russell Lee Deter
Book Title : Fetal Growth Restriction
Book Subtitle : Current Evidence and Clinical Practice
Editors : Luciano Marcondes Machado Nardozza, Edward Araujo Júnior, Giuseppe Rizzo, Russell Lee Deter
DOI : https://doi.org/10.1007/978-3-030-00051-6
Publisher : Springer Cham
eBook Packages : Medicine , Medicine (R0)
Copyright Information : Springer Nature Switzerland AG 2019
Hardcover ISBN : 978-3-030-00050-9 Published: 04 January 2019
eBook ISBN : 978-3-030-00051-6 Published: 14 December 2018
Edition Number : 1
Number of Pages : XV, 238
Number of Illustrations : 6 b/w illustrations, 41 illustrations in colour
Topics : Obstetrics/Perinatology/Midwifery , Imaging / Radiology
Policies and ethics
- Find a journal
- Track your research
Selective Fetal Growth Restriction: What We Know and What We Don’t
Please note:.
This course may contain imagery and/or video of surgical procedures.
In this session, learners will be able to identify maternal and fetal indications for various fetal interventions and associated maternal-fetal outcomes and discuss prenatal counseling, obstetrical management, fetal therapy options, fetal surveillance and delivery planning for fetuses with select fetal anomalies. Learners will also be able to understand ongoing research endeavors related to maternal-fetal health and childhood outcomes.
- Nahla Khalek, MD, MPH, FACOG, MSEd
- Shelly Soni, MD
- Juliana Gebb, MD
This seminar focuses on fetal diagnosis and treatment and was delivered at a virtual event titled, “Selective Fetal Growth Restriction: What We Know and What We Don’t” on December 8, 2023.
Additional CHOP Resources:
- Richard D. Wood Jr. Center for Fetal Diagnosis and Treatment
- Upcoming Healthcare Professionals Events
Content Disclaimer
The Terms of Use and Privacy Policy set forth on the website of The Children’s Hospital of Philadelphia apply to any and all uses of and access to this site and the content found here.
The work presented in the presentations, videos, and other content on this site (“Presentations”) includes publicly available medical evidence, a consensus of medical practitioners, and/or opinions of individual practitioners that may differ from consensus opinions. These Presentations are intended only to provide general information and need to be adapted for each specific patient based on the practitioner’s professional judgment, consideration of any unique circumstances, the needs of each patient and their family, the availability of various resources at the health care institution where the patient is located, and other factors. The Presentations are not intended to constitute medical advice or treatment, nor should they be relied upon as such. The Presentations are not intended to create a doctor-patient relationship between/among The Children’s Hospital of Philadelphia, its physicians and the individual patients in question. The information contained in these Presentations are general in nature, and do not and are not intended to refer to specific patients.
CHOP, The Children’s Hospital of Philadelphia Foundation and its or their affiliates, the authors, presenters, practitioners, editors, and others associated with the creation of the Presentations (“CHOP”) are not responsible for errors or omissions in the Presentations; for any outcomes a patient might experience where a clinician reviewed one or more such Presentations in connection with providing care for that patient; and/or for any and all third party content on the site or in the Presentations. CHOP makes no warranty, expressed or implied, with respect to the currency, completeness, applicability or accuracy of the Presentations. Application of the information in or to a particular situation remains the professional responsibility of the practitioner who is directly treating the patient.
To the extent that the Presentations include information regarding drug dosing, in view of ongoing research, changes in government regulations and the constant flow of information relating to drug therapy and drug reactions, the viewer should not rely on the Presentation content, but rather is urged to check the package insert for each drug for indications, dosage, warnings and precautions.
Some drugs and medical devices presented in the Presentations have United States Food and Drug Administration (FDA) clearance for limited use in restricted research settings. It is the responsibility of the practitioner to ascertain the FDA status of each drug or device planned for use in their clinical practice.
You shall indemnify, defend and hold harmless CHOP, The Children’s Hospital of Philadelphia Foundation, and its/their current and former employees, officers, and agents, trustees, and their respective successors, heirs and assigns (“Indemnitees”) against any claims, liability, damage, loss or expenses (including attorneys’ fees and expenses of litigation) in connection with any claims, suits, actions, demands or judgments arising directly or indirectly out of your reference to or use of the Presentations.
The Presentations are protected by copyright laws and in some cases patent laws, and all rights are reserved under such laws. No part of the Presentations may be reproduced in any form by any means, or utilized in any other way, absent prior written permission from the copyright owner.
By starting this module, you agree to our Content Disclaimer and Terms of Service.
Course Content
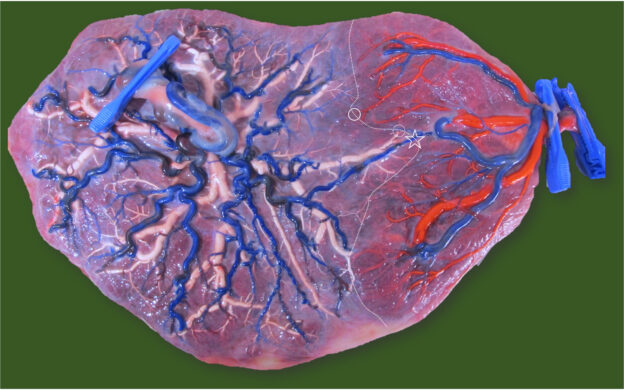
Course Includes
- Course Certificate
CHOP Employee? Log In Here
Username or Email Address
Forgot Password?
Remember Me

An official website of the United States government
The .gov means it’s official. Federal government websites often end in .gov or .mil. Before sharing sensitive information, make sure you’re on a federal government site.
The site is secure. The https:// ensures that you are connecting to the official website and that any information you provide is encrypted and transmitted securely.
- Publications
- Account settings
Preview improvements coming to the PMC website in October 2024. Learn More or Try it out now .
- Advanced Search
- Journal List
- Geburtshilfe Frauenheilkd
- v.80(10); 2020 Oct
Fetal Growth Restriction – Diagnostic Work-up, Management and Delivery
Fetale wachstumsrestriktion – diagnostik, betreuung und entbindung, dietmar schlembach.
Vivantes – Netzwerk für Gesundheit GmbH, Klinikum Neukölln, Klinik für Geburtsmedizin, Berlin, Germany
Fetal or intrauterine growth restriction (FGR/IUGR) affects approximately 5 – 8% of all pregnancies and refers to a fetus not exploiting its genetically determined growth potential. Not only a major cause of perinatal morbidity and mortality, it also predisposes these fetuses to the development of chronic disorders in later life. Apart from the timely diagnosis and identification of the causes of FGR, the obstetric challenge primarily entails continued antenatal management with optimum timing of delivery. In order to minimise premature birth morbidity, intensive fetal monitoring aims to prolong the pregnancy and at the same time intervene, i.e. deliver, before the fetus is threatened or harmed. It is important to note that early-onset FGR (< 32 + 0 weeks of gestation [wks]) should be assessed differently than late-onset FGR (≥ 32 + 0 wks). In early-onset FGR progressive deterioration is reflected in abnormal venous Doppler parameters, while in late-onset FGR this manifests primarily in abnormal cerebral Doppler ultrasound. According to our current understanding, the “optimum” approach for monitoring and timing of delivery in early-onset FGR combines computerized CTG with the ductus venosus Doppler, while in late-onset FGR assessment of the cerebral Doppler parameters becomes more important.
Introduction
Growth of the healthy fetus usually follows a linear pattern, i.e., with constant percentile growth, thus exploiting its genetically determined growth potential. A fetus diagnosed to be small at ultrasound requires a structured diagnostic work-up in order to achieve an optimal ante- and perinatal management.
Fetal or intrauterine growth restriction (FGR/IUGR) affects approximately 5 – 8% of all pregnancies and refers to a fetus not exploiting its genetically determined growth potential. Presently, FGR is classified into early (early-onset < 32 + 0 weeks of gestation [wks]) and late FGR (late-onset ≥ 32 + 0 wks) 1 . FGR is one of the main causes of perinatal morbidity and mortality, and this is especially true when fetal growth problems are not recognised as such before delivery 2 . Moreover, fetal growth restriction apparently predisposes to the development of chronic disorders in later life 3 , 4 , 5 , 6 .
The percentage of fetuses with FGR due to (relative) uteroplacental dysfunction and/or concomitant relative maternal heart failure increases particularly in late and prolonged pregnancy, and this is associated with a corresponding perinatal risk 7 .
Apart from the timely diagnosis and identification of the causes of FGR, the obstetric challenge primarily entails continued antenatal management with optimum timing of delivery. Intensive fetal monitoring aims to prolong the pregnancy in order to minimise preterm morbidity, and at the same time to intervene, i.e. deliver, before the fetus is threatened or harmed. This review summarises the current recommendations of the German AWMF guideline 015/080 “Intrauterine Growth Restriction” 1 .
Definitions
The definitions of constitutionally small fetuses and those with fetal growth restriction in international guidelines and literature vary greatly 8 . In particular, the terms “small for gestational age” (SGA) and FGR must be differentiated with regard to content and thus clinical management. In line with the guidelines, this review uses the terms SGA and FGR (IUGR) solely in terms of fetal growth and does not consider any other fetal conditions.
SGA fetuses consistently demonstrate growth rates below the 10th percentile 1 . In many cases this is more a reflection of constitutional factors such as gender, parental height and ethnicity and is usually not linked to a medical condition. SGA fetuses continue to grow linearly and do not exhibit other parameters of fetal distress (e.g., oligohydramnios or Doppler abnormalities); however, it should be noted that the lower the percentile, the higher the morbidity and mortality risk: SGA fetuses with growth below the 3rd percentile have a significantly higher morbidity and mortality risk despite constant percentile growth 9 .
SGA fetus must be differentiated from FGR, since in the latter cases the fetus does not realise its genetically determined growth potential. This results in the typical flattened growth curve, i.e., a “crossing of centiles”. Often FGR fetuses also demonstrate growth below the 10th percentile, i.e., they are “small for gestational age”, but a flattened growth curve (and thus FGR) may also appear in fetuses with an estimated weight above the 10th percentile, especially in third trimester late-onset FGR.
FGR is one of the most common causes of obstetric complications with unfavourable perinatal and neonatal outcome, particularly in the context of prematurity. The fetuses in question exhibit a higher prevalence of poor long-term neurological development as well as cardiovascular and endocrinological disorders 3 , 4 , 5 , 6 . Almost 30 – 50% of all intrauterine deaths are related to FGR 10 .
According to current expert opinion, fetal abdominal circumference or estimated fetal weight < 3rd percentile and abnormal indices of the umbilical artery are decisive parameters for the definition of early-onset or late-onset FGR 11 . According to the current German guideline, the following definitions apply to SGA and FGR (see box) 1 :
Aetiology and Epidemiology
The pathogenesis of fetal hypotrophy includes maternal, fetal and placental factors ( Table 1 ) 1 . In many cases, the different underlying pathophysiological mechanisms ultimately culminate in placental failure, which occurs as a result of unsuitable transformation of the maternal spiral arteries due to inadequate invasion of extravillous trophoblasts and thus deficient uterine perfusion. This leads to placental hypoxia with secondary injury to the villous architecture 12 .
Table 1 Causes and risk factors of fetal growth restriction (according to 1 ).
Diagnostic Work-up
Prenatal management according to the maternity guidelines includes regular monitoring of the fundal height. Since the available clinical examinations can only provide limited information, the diagnostic work-up should be extended (initially by ultrasound) in case of abnormalities, i.e., “abdominal circumference too small/fundal height too low”. Fig. 1 summarises the examination algorithm in suspected SGA/FGR.

Diagnostic examination algorithm in suspected SGA/FGR.
Checking the gestational age
The diagnosis of FGR includes the most accurate assessment of the gestational age possible. Ideally, this is based on the crown-rump length (CRL) in the first trimester. This parameter provides the most reliable information 13 ; in case of discrepancies in an otherwise unremarkable embryo, the (anamnestic) gestational age should be corrected by ultrasound, if it differs by at least 7 days from the age determined by ultrasonography – unless the date of conception is definitely known (e.g. IVF/ICSI) 1 .
If CRL measurements are not available (e.g., if pregnancy is diagnosed late), the gestational age may also be estimated from the biparietal or transcerebellar diameter.
A discrepancy between the gestational age calculated based on the last period and according to ultrasound may be the first sign of an early developmental disorder. Such cases call for further assessment and intensive monitoring.
Apart from estimated weight, fetal abdominal circumference is the most important indicator of impaired fetal growth. Fetal weight can be determined with the Hadlock formula, which is recommended in increased risk of FGR 1 . It should be noted that parental characteristics need to be taken into account when estimating weight.
According to the definition of FGR, assessment should include not only the current estimated weight, but also the growth curve in order to detect flattening of the latter, especially since FGR is not limited to weights below the 10th percentile.
Amniotic fluid
Amniotic fluid volume is often reduced in FGR, as this disorder can be accompanied by fetal oliguria. But usually the amniotic fluid volume is unremarkable 1 , 14 . Evaluation of the amniotic fluid volume may be based on the “single deepest pocket” (SDP) technique or measurement of the amniotic fluid index (AFI) 15 , with the SDP appearing to be more useful in predicting adverse outcome 16 .
If the suspected diagnosis of SGA/FGR is confirmed, this should be followed by further evaluation of the causes and assessment of the fetal condition. This includes Doppler ultrasound assessment of the uteroplacental unit (uterine and umbilical arteries) and possibly other fetal vessels (middle cerebral artery, ductus venosus), as well as detailed diagnostic ultrasonography. In addition, differential diagnosis may profit from genetic assessment of any chromosomal pathology and/or fetal infection when planning the subsequent management 1 .
Detailed diagnostic ultrasonography
Differential diagnostic assessment of possible FGR calls for further differentiated diagnostic organ work-up (along the lines of the German DEGUM II criteria) ( Fig. 1 ) 1 . It should be noted that structural abnormalities of the fetus are indicative of genetic-syndromal diseases, especially in early-onset and multiple pathologies 17 . At least the genetic analysis should be offered.
Doppler ultrasound
Doppler ultrasound is mandatory in suspected SGA/FGR, not only for the differential diagnosis of SGA/FGR, but also for determining the cause of the FGR. Higher indices in the uterine and umbilical arteries indicate placental disorder along the lines of placental failure. When diagnosing FGR, other fetal vessels (middle cerebral artery, ductus venosus) should be examined as well to evaluate the fetal condition 1 .
Screening, Prediction and Prevention
At present, there is no screening approach available combining good sensitivity and specificity with negative or positive predictive value 18 .Similar to the first-trimester risk evaluation in pre-eclampsia, screening for fetal growth restriction can be performed by combining the maternal medical history, Doppler ultrasound of the uterine arteries, mean arterial blood pressure, and the biochemical marker PAPP-A 19 . Even if the detection rate does not match that of pre-eclampsia screening, it can be used to detect some pregnancies with a high risk of FGR which will then be closely monitored.
In the second trimester Doppler ultrasound of the uterine arteries in low-risk cohorts is only of limited use, while in a cohort at risk it offers a moderate predictive benefit for early detection of FGR 20 . Therefore, Doppler ultrasound of the uterine arteries when screening for FGR is regarded as somewhat under discussion 21 . However, abnormal findings should prompt regular ultrasound studies of growth and Doppler checks (uterine and umbilical arteries).
The combination of Doppler ultrasound and angiogenic factors (e.g., sFlt-1/PlGF ratio) appears to improve the FGR prediction, as does the combination of fetal biometry and the angiogenic marker 22 , 23 , 24 . However, further studies are still needed before widespread clinical use.
Antenatal diagnosis of FGR is essential, since this has a positive effect not only on the course of pregnancy but also on neonatal outcome 2 , 25 . Despite closely monitored management internationally and also in Germany, the number of unidentified antenatal FGR cases is still large 1 , 25 .
According to maternity guidelines, fetometry is performed between 18 + 0 and 21 + 6 wks and 28 + 0 and 31 + 6 wks. This approach detects early-onset FGR quite well, but does not identify the majority of late-onset growth restrictions (approx. 70 – 80% of FGR), particularly if no Doppler ultrasound evaluation or subsequent biometry is performed.
In order to improve this situation, the definition of FGR requires that the assessment should include not only the current estimated weight, but also the growth curve in order to detect flattening of the latter, especially since FGR is not limited to weights below the 10th percentile. In case of irregularities, further studies, i.e., repeat biometry, Doppler ultrasound and possibly measurement of the angiogenic markers could be performed 26 . This is particularly important, since early/correct diagnosis of FGR can reduce the perinatal risk 27 .
An (additional) late biometry at ≥ 36 wks improves the FGR detection rate by a factor of 3 28 , 29 . Combined with the assessment of fetal growth over time it is possible to detect a subgroup with high perinatal risk 28 , 30 . Pathologies on Doppler ultrasound (maternal, fetoplacental or fetal) correlate with less favourable perinatal outcome 27 , 31 , 32 . 15 – 20% of cases with late-onset FGR exhibit abnormal cerebral perfusion – with unremarkable blood flow in the umbilical cord; by determining the cerebroplacental ratio (CPR) fetuses (with and without growth problems) at increased risk of unfavourable perinatal outcome can be detected more easily 33 , 34 , 35 . The combination of biometry, uterine Doppler ultrasound and CPR in the third trimester can detect the majority of fetuses with a high risk of intrauterine fetal death (IUFT) 31 , but appears to be of little help in detecting SGA/FGR in non-selected populations 36 . The combination of fetal biometry and measurement of angiogenic factors (especially the sFlt-1/PlGF ratio) also seems to be useful in FGR detection 23 , 37 , 38 .
Unlike in pre-eclampsia, the administration of low-dose aspirin appears to be only moderately successful in the prevention of FGR (although the trial did not have enough statistical power for the prevention of FGR) 39 ; this also requires further studies. The results of the German multicentre trial on the benefit of NO-donor PETN in women with pathological Doppler ultrasound of the uterine arteries in the second trimester are expected soon 40 .
SGA/FGR Management
FGR management is a challenge for all involved: Fetal hypoxemia should be diagnosed early to avoid irreversible damage and intrauterine death. On the other hand, in order to minimise the sequelae of prematurity pregnancy should not be terminated too early.
Parents must be involved in all decisions and the consequences of the different options must be explained to them. In particular, the increased risk of intrauterine fetal death under “watchful waiting” must be contrasted with the increased mortality and morbidity risk of prematurity at delivery. Thus, close antenatal and perinatal cooperation with the neonatologists is necessary to provide the parents with adequate information.
It is important to note that early-onset FGR should be assessed differently than late-onset FGR. In early-onset FGR progressive deterioration is reflected in abnormal venous Doppler parameters, while in late-onset FGR this manifests primarily in cerebral Doppler sonography ( Table 2 ) 1 , 41 .
Table 2 Early-/late-onset FGR (according to 42 ).
No individual monitoring approach can predict the outcome of FGR in valid fashion; a combination of different approaches is recommended. In order to improve the perinatal outcome, FGR monitoring requires in particular ultrasonography and above all Doppler ultrasound.
Management of pregnancies with SGA or FGR fetuses relies on a combination of different examination techniques, which are summarised in Fig. 2 1 . Monitoring of fetal growth, amniotic fluid volume, and arterial and venous fetal vessels is mandatory; CTG/computerised CTG monitoring also provides important information on the fetal condition 1 , 42 .

Management protocol in fetal growth restriction (data from 1 ).
Biometric monitoring
Serial ultrasound monitoring of fetal growth is essential in the management of fetuses with SGA/FGR. However, it should be noted that – also due to the limitations of weight estimation by ultrasonography – the intervals between examinations should not be too short: the interval between such examinations should be at least 2, preferably 3 weeks 1 , 43 .
- Umbilical artery: Doppler ultrasound of the umbilical arteries permits haemodynamic changes to be detected in the course of the disease. In FGR normal resistance ratios also indicate a low risk of unfavourable perinatal outcome and low perinatal mortality 9 . The prognosis worsens depending on the degree of increase in resistance 41 . In so-called ARED flow (absent/reversed end-diastolic flow) about 70% of the placental vessels are occluded 44 , which in “reversed end-diastolic flow” increases the risk of perinatal mortality by a factor of 5. Fig. 2 lists the recommended study intervals based on studies of progressive Doppler deterioration 45 . If the resistance in the umbilical arteries is unremarkable, two-week intervals appear to be adequate 46 .
- Ductus venosus: In the care of early-onset FGR the ductus venosus is a key vessel 41 , and management of early-onset FGR should base its assessment on the evaluation of this vessel 1 . Changes in the venous circulation including reversed blood flow usually appear later than changes in the arterial system 41 . Pathophysiologically, increasing cardiac dysfunction results in decreased diastolic blood flow or increased pulsatility in the ductus venosus including loss of the positive a-wave; absent a-wave or reverse blood flow is an indication of cardiovascular instability and may be a sign of imminent or already present acidemia 47 , 48 , 49 , with the risk of intrauterine death doubling daily 45 , 47 .
- Middle cerebral artery: Examination of the middle cerebral artery (MCA) can detect progressive hypoxaemia, since the increasing fetal compromise leads to a redistribution of blood (“brain-sparing effect”) 41 . MCA resistance decreases and is considered abnormal for a pulsatility index (PI) < 5th percentile 1 . While in early-onset FGR the predictive power of the MCA is limited with regard to predicting unfavourable perinatal outcome 50 , 51 , 52 , the examination of the MCA, preferably when combined with examination of the umbilical artery as the so-called cerebroplacental ratio (CPR), gains importance in the management of late-onset FGR. On the one hand, CPR allows a more precise diagnosis of late-onset FGR 42 , while various studies have demonstrated a benefit in predicting unfavourable perinatal outcomes in pathological CPR, i.e., ratios between umbilical artery and and middle cerebral artery (< 5th percentile or < 1) 53 , 54 , 55 , 56 .
(Computerised) Cardiotocography (cCTG)
According to maternity guidelines cardiotocography (CTG) should always be performed as part of prenatal care if placental insufficiency is suspected 1 . However, it detects acute rather than chronic courses 41 and therefore should never be the sole monitoring technique when managing FGR 1 .
To minimize the limitations of a CTG examination (subjective assessment), a computer-based analysis of the CTG can be employed. Major benefits of this technique include the higher degree of objectivity of the assessment as well as the option of analysing the short-term variation (STV) 57 . As in uncomplicated pregnancies, the STV increases with gestational age in FGR, but the STV values are generally lower 58 . An STV of 4.5 ms rules out fetal acidemia (NPV 100%) 59 , while decreasing STV values are associated with earlier delivery, lower birth weight, lower pH of the umbilical artery, poorer acid-base status and worse neonatal outcome 60 .
Measuring the STV and observing its course over time may detect subtle changes, which can be helpful for better timing of the delivery 61 , thus underlining the use of this measure in FGR management; nevertheless, it must be taken into account that CTG changes in FGR only manifest themselves rather late 41 , 61 , and that the short-term variation is also affected by medication (e.g., with antenatal corticosteroids), which must therefore be considered when interpreting the results 1 , 62 , 63 .
Other Measures
Inpatient/outpatient management.
In general, surveillance of pregnancies with FGR may be performed in an outpatient setting, as there is no evidence-based data on which to base indications for inpatient monitoring. With increasing severity and fetal impairment, however, inpatient monitoring can be helpful if close intervals between examinations become necessary. The link between early-onset FGR and pre-eclampsia should also be noted. The decision for outpatient or inpatient care should be made on a case by case basis 1 .
Antenatal corticosteroid administration
One challenge in obstetrics (and thus also in the management of FGR) is in estimating the time of expected delivery in the preterm setting. If delivery is expected within the next 7 days, corticosteroids should be administered 1 , 64 . The benefits of antenatal corticosteroids are also seen in growth-restricted fetuses. It should be noted, however, that corticosteroid administration may temporarily reduce heart rate variation as well as fetal body and respiratory movements; these changes normalise within 72 hours 1 .
In addition to the “timing” of steroid administration noted above, it generally is true that non-critical administration of steroids should not be undertaken in all FGRs, since corticosteroids – in addition to their undisputed positive benefits – also have various negative effects (including reduced growth, delayed neurological development, stress, hypertension). In addition, fetuses with FGR, especially with increasing hypoxaemia, already possess high cortisol levels, and after 30 wks fetuses with FGR develop RDS much less often than eutrophic fetuses. There is insufficient data on the effect of steroids on long-term outcome in fetuses > 30 wks with FGR.
Therefore, the goal should be to avoid as much as possible antenatal steroid application, especially repeated courses in women who in the end do not give premature birth 65 .
Magnesium for fetal neuroprotection
The antenatal intravenous administration of magnesium appears to have a neuroprotective effect and thus may help to reduce the risk of neurological damage. Since FGR correlates with an increased risk of fetal prematurity, (inter)national medical societies recommend the administration of magnesium sulphate before 32 + 0 wks for neuroprotection when birth is imminent 1 , 64 .
Birth/Delivery in SGA/FGR
Place of delivery.
In order to ensure immediate and continuous care in the FGR setting, delivery should proceed in a perinatal centre with neonatal intensive care unit and an experienced paediatric team 1 .
Time of delivery
When planning the timing of delivery, the risks of preterm delivery must be weighed against those of continuing the pregnancy and/or the maternal risks. Maternal delivery criteria apply regardless of the gestational age and extent of the FGR.
In terms of the fetus, the timing of delivery is governed not only by the gestational age but also the Doppler ultrasound findings ( Fig. 2 ). As noted above, early-onset FGR is accompanied by a serious deterioration, particularly in venous abnormalities (ductus venosus), while late-onset FGR is associated with cerebral vascular disorders (ACM, CPR). This is reflected in the recommendations of (inter)national guidelines and experts 1 , 42 , 66 , 67 .
Note: Just like decelerations, the cut-off values (cCTG and ductus venosus) given below are late signs of fetal deterioration. Some of these cases may already suffer from myocardial dysfunction and possibly hypotension, and the fetal adaptation mechanisms no longer fulfil their protective function. This implies that the significance of these parameters diminishes with increasing gestational age.
- cCTG: In case of new-onset CTG pathologies (recurrent, unprovoked, and refractory decelerations at any time) delivery should be considered 1 , 61 . Delivery should be considered if STV is < 2.6 ms at 26 + 0 to 28 + 6 wks and < 3.0 ms at 29 + 0 to 32 + 0 wks ( Fig. 2 ) 1 , 61 .
- Ductus venosus (early-onset FGR): According to current knowledge, pathological findings of the ductus venosus may be an indication for delivery if the fetus is viable and antenatal corticosteroids have been administered 1 . Depending on the gestational age and possible additional findings, delivery should be discussed with the expectant mother in case of increased resistance (> 95th percentile – accompanied by a decreased a-wave); in case of absent or reverse a-waves delivery is indicated 1 , 41 , 42 , 61 , 66 .
According to our current understanding, the combination of computerised CTG and ductus venosus is the “optimum” approach for monitoring early-onset FGR 68 . If Doppler ultrasound of the ductus venosus is unremarkable and in the absence of cCTG pathology, it may nevertheless be appropriate to terminate the pregnancy earlier.
- Umbilical artery: With absent or reversed end-diastolic flow (AREDF) in the umbilical artery the prognosis can be poor 1 , 41 , 69 , but the morbidity and mortality associated with preterm birth before 32 + 0 wks is also rather high 70 , while continued pregnancy offers clear benefits in outcome 71 . Taking this into account, for ARED-flow the current guideline recommends: In REDF, delivery may be considered from 30 + 0 wks and should be performed at 32 + 0 wks. In absent end-diastolic flow (AEDF) the mortality risk is lower, but delivery should be performed at 34 + 0 wks 1 . In an otherwise unremarkable course, waiting until these weeks of pregnancy is possible and after two years does not result in any significant differences in morbidity and mortality 72 . Increased resistance in the umbilical artery (PI > 95th percentile) is also linked to increased risk in perinatal morbidity and mortality, but with low predictive power. With increased PI > 95th percentile, delivery is therefore recommended from 37 + 0 wks 1 .
- Middle cerebral artery/cerebroplacental ratio: In the preterm setting (< 37 + 0 wks), the prognostic power of the MCA is of limited use in predicting acidemia and poor perinatal outcome, and should not be used to determine delivery timing at this stage 1 . Delivery should be considered starting at 37 + 0 wks if resistance in the MCA is low (PI < 5th percentile) 1 . At present, the cerebroplacental ratio (CPR) is still under contention, since precise limits in particular have not been clearly evaluated yet. Since some studies have shown that an abnormally low CPR is a predictor of poor perinatal outcome, delivery can be considered from 37 + 0 wks 1 .
Since fetuses with isolated SGA, i.e. fetal growth < 10th percentile, unremarkable Doppler findings and no additional risks, have an increased risk of intrauterine death, despite a generally favourable outcome, earlier delivery from 38 + 0 wks may be considered in SGA fetuses as well. In fetuses with isolated SGA prolonged pregnancy should be avoided 1 , because ultimately SGA and GFR cannot be reliably differentiated with current techniques of fetal monitoring (biometry, utero-umbilico-fetal Doppler, BPS, NST) in all cases, and relative uteroplacental failure is increasingly seen close to the expected date of delivery.
When Doppler ultrasound and cCTG are unremarkable, isolated growth arrest is not an independent factor for immediate termination of pregnancy. These constellations should always consider the gestational age and the measurement interval should be checked in order to minimise the systematic error when estimating the weight by ultrasonography 1 .
Type of delivery
In addition to gestational age, parity and cervical maturation, various other factors such as the presence of abnormal findings (Doppler, cCTG) and other fetal or maternal specifics or complications must be taken into account when deciding on the type of delivery, and this decision must be made for each patient individually 1 :
In FGR with unremarkable Doppler findings or increased pulsatility in the umbilical artery (> 95th percentile) – not with ARED flow – labour can be induced and vaginal delivery attempted. However, the higher risk of complications must be taken into account mandating continuous intrapartum monitoring 1 .
In abnormal Doppler findings such as increased resistance in the umbilical artery (not ARED flow) and in late-onset FGR with abnormal ACM/CPR values, induction of labour and vaginal delivery are possible, with continuous intrapartum monitoring mandatory 1 .
In early-onset FGR with pathological cCTG, abnormal ductus venosus and/or especially in ARED-flow, caesarean section is usually recommended and performed, if only because of the increasingly impaired fetal condition that this situation signifies. Also in very early weeks of pregnancy caesarean section must be performed if the termination of pregnancy is indicated and when there is no meaningful option to induce delivery 1 .
Acknowledgements
I would like to thank the expert authors of the AWMF guideline on IUGR, on which this review is based.
Mein Dank gilt der Expertengruppe, die die AWMF-Leitlinie IUGR erstellt hat und auf der diese Arbeit basiert.
Conflict of Interest/Interessenkonflikt The authors declare that they have no conflict of interest./Die Autorinnen/Autoren geben an, dass kein Interessenkonflikt besteht.
SGA/FGR definition according to AWMF Guideline 015/080
- Estimated fetal weight or birth weight < 10th percentile
- Estimated fetal weight < 10th percentile and/or
- “crossing of centiles” growth and
- Abnormal umbilical artery Doppler ultrasound or
- Abnormal uterine artery Doppler ultrasound or
- Oligohydramnios
References/Literatur
- Geburtshilfe Frauenheilkd. 2020 Oct; 80(10): 1016–1025.
Zusammenfassung
Die fetale oder intrauterine Wachstumsrestriktion (FGR/IUGR) tritt in ca. 5 – 8% aller Schwangerschaften auf und definiert einen Fetus, der sein genetisch vorgegebenes Wachstumspotenzial nicht ausschöpft. Sie stellt einen Hauptgrund der perinatalen Morbidität und Mortalität dar und ist zudem mit einer Prädisposition für die Entwicklung chronischer Erkrankungen im weiteren Leben assoziiert. Die geburtshilfliche Herausforderung stellt neben der rechtzeitigen Diagnose und der Ursachenklärung einer FGR vor allem die weitere Schwangerschaftsbetreuung mit der Wahl des optimalen Entbindungszeitpunkts dar. Ziel einer intensiven fetalen Überwachung ist es, eine Schwangerschaftsprolongation zur Minimierung der Frühgeburtsmorbidität zu erreichen, aber rechtzeitig vor einer fetalen Bedrohung oder Schädigung zu intervenieren, d. h. zu entbinden. Zu beachten ist, dass eine frühe FGR (< 32 + 0 SSW) und eine späte FGR (≥ 32 + 0 SSW) unterschiedlich beurteilt werden sollen. Eine zunehmende Verschlechterung spiegelt sich bei einer frühen FGR in Auffälligkeiten venöser Doppler-Parameter wider, bei einer späten FGR vor allem in der zerebralen Doppler-Sonografie. Die „optimale“ Methode zur Überwachung und Entscheidung zur Entbindung stellt bei einer frühen FGR nach derzeitigem Kenntnisstand die Kombination von computerisiertem CTG und Ductus venosus dar, bei einer späten FGR tritt die Beurteilung der zerebralen Doppler-Parameter in den Vordergrund.
Der gesunde Fetus wächst normalerweise linear, d. h. perzentilenkonstant und schöpft somit sein genetisch vorgegebenes Wachstumspotenzial aus. Ein sonografisch kleiner Fetus erfordert ein strukturiertes diagnostisches Vorgehen für eine optimale prä- und peripartale Betreuung der Schwangeren.
Die fetale oder intrauterine Wachstumsrestriktion (FGR/IUGR) tritt in ca. 5 – 8% aller Schwangerschaften auf und definiert einen Fetus, der sein genetisch vorgegebenes Wachstumspotenzial nicht ausschöpft. Aktuell wird zwischen der frühen (early-onset < 32 + 0 SSW) und der späten (late-onset ≥ 32 + 0 SSW) FGR unterschieden 1 . Die FGR stellt einen Hauptgrund der perinatalen Morbidität und Mortalität dar; dies gilt insbesondere dann, wenn eine Wachstumsproblematik des Fetus pränatal nicht als solche erkannt wird 2 . Darüber hinaus ist eine fetale Wachstumsrestriktion mit einer Prädisposition für die Entwicklung chronischer Erkrankungen im weiteren Leben assoziiert 3 , 4 , 5 , 6 .
Durch (relative) uteroplazentare Dysfunktion und durch eine zusätzliche relative maternale Herzinsuffizienz nimmt insbesondere in der Spätschwangerschaft und vor allem bei Terminüberschreitung der Anteil von FGR-Feten zu, was mit einer entsprechenden perinatalen Gefährdung verbunden ist 7 .
Die geburtshilfliche Herausforderung stellt neben der rechtzeitigen Diagnose und der Ursachenklärung einer FGR vor allem die weitere Schwangerschaftsbetreuung mit der Wahl des optimalen Entbindungszeitpunkts dar. Ziel einer intensiven fetalen Überwachung ist es, eine Schwangerschaftsprolongation zur Minimierung der Frühgeburtsmorbidität zu erreichen, aber rechtzeitig vor einer fetalen Bedrohung oder Schädigung zu intervenieren, d. h. zu entbinden. Dieser Artikel fasst die aktuellen Empfehlungen der deutschsprachigen AWMF-Leitlinie 015/080 „Intrauterine Wachstumsrestriktion“ zusammen 1 .
Definitionen
Die Definitionen für konstitutionell kleine und Feten mit einer fetalen Wachstumsrestriktion sind in internationalen Leitlinien und Literatur sehr heterogen 8 . Es gilt, insbesondere die Begriffe „small for gestational age“ (SGA) und FGR inhaltlich und folglich im klinischen Management zu unterscheiden. Die hier vorliegende Arbeit nutzt die Begriff SGA bzw. FGR (IUGR) leitlinienkonform ausschließlich mit Hinblick auf das fetale Wachstum und berücksichtigt nicht evtl. zusätzliche fetale Erkrankungen.
SGA-Feten weisen ein perzentilenkonstantes Wachstum unter der 10. Perzentile auf 1 . Dies ist oft ein Ausdruck konstitutioneller Faktoren wie beispielsweise Geschlecht, Größe der Eltern und Ethnizität und geht meist nicht mit einer Pathologie einher. SGA-Feten wachsen weiterhin linear und zeigen keine sonstigen Zusatzparameter für fetalen Distress (z. B. Oligohydramnion oder Doppler-Auffälligkeiten); allerdings gilt zu beachten, dass je geringer die Perzentile ist, desto höher ist das Morbiditäts- und Mortalitätsrisiko: SGA-Feten mit einem Wachstum unterhalb der 3. Perzentile weisen trotz perzentilenkonstantem Wachstum ein signifikant höheres Morbiditäts- und Mortalitätsrisiko auf 9 .
Abzugrenzen von einem SGA-Fetus ist eine FGR, da in diesen Fällen der Fetus sein genetisch vorgegebenes Wachstumspotenzial nicht erreicht. Typischerweise kommt es hier zur Wachstumsabflachung, d. h. einem „crossing of centiles“. Oft weisen FGR-Feten auch ein Wachstum unterhalb der 10. Perzentile auf, sind also „small for gestational age“; eine Wachstumsabflachung (und somit FGR) kann aber auch bei Feten mit einem Schätzgewicht über der 10. Perzentile auftreten, insbesondere bei der spät einsetzenden FGR im 3. Trimenon.
Die FGR ist eine der häufigsten Ursachen für geburtshilfliche Komplikationen mit ungünstigem perinatalen und neonatalen Outcome, insbesondere im Zusammenhang mit Frühgeburtlichkeit. Die betroffenen Feten zeigen eine höhere Prävalenz für eine schlechtere neurologische Langzeitentwicklung sowie für kardiovaskuläre und endokrinologische Störungen 3 , 4 , 5 , 6 . Nahezu 30 – 50% aller intrauterinen Fruchttode sind mit einer FGR assoziiert 10 .
Ein fetaler Abdomenumfang oder ein fetales Schätzgewicht < 3. Perzentile und pathologische Indices der A. umbilicalis sind nach derzeitiger Expertenmeinung entscheidende Parameter für die Definition einer frühen oder späten FGR 11 . Nach der derzeit gültigen deutschsprachigen Leitlinie gelten die folgenden Definitionen für SGA bzw. FGR (siehe Kasten) 1 :
Definition SGA/FGR nach der AWMF-Leitlinie 015/080
- fetales Schätzgewicht oder Geburtsgewicht < 10. Perzentile
- fetales Schätzgewicht < 10. Perzentile und/oder
- nicht perzentilengerechtes Wachstum im Verlauf („crossing of centiles“) und
- pathologische Doppler-Sonografie der A. umbilicalis oder
- pathologische Doppler-Sonografie der Aa. uterinae oder
- Oligohydramnion
Ätiologie und Epidemiologie
Die Genese der fetalen Hypotrophie umfasst maternale, fetale und plazentare Faktoren ( Tab. 1 ) 1 . Die zugrunde liegenden unterschiedlichen pathophysiologischen Mechanismen münden letztendlich oft in eine Plazentainsuffizienz, die infolge einer unzureichenden Umwandlung der maternalen Spiralarterien durch inadäquate Invasion extravillöser Trophoblastzellen und damit mangelhafter uteriner Perfusion auftritt. Es kommt zur plazentaren Hypoxie mit sekundärer Schädigung der Zottenarchitektur 12 .
Tab. 1 Ursachen und Risikofaktoren einer fetalen Wachstumsrestriktion (nach 1 ).
Im Rahmen der Schwangerenvorsorge nach den Mutterschaftsrichtlinien erfolgt eine regelmäßige Kontrolle des Fundusstandes. Da die zur Verfügung stehenden klinischen Untersuchungen in ihrer Aussagekraft limitiert sind, soll bei Auffälligkeiten, d. h. „zu kleinem Bauch/zu niedrigem Fundusstand“ eine weitere Diagnostik (zunächst mittels Ultraschall) erfolgen. Abb. 1 fasst die Untersuchungsalgorithmus bei V. a. SGA/FGR zusammen.

Untersuchungsalgorithmus zur Abklärung bei V. a. SGA/FGR.
Überprüfung des Gestationsalters
Zur Diagnose einer FGR gehört die möglichst annähernd korrekte Bestimmung des Gestationsalters. Idealerweise wird hierzu die Scheitelsteißlänge (SSL) im 1. Trimenon herangezogen. Dieses Verfahren liefert die verlässlichsten Angaben 13 , bei Diskrepanzen soll bei sonografisch ansonsten unauffälligem Embryo – außer bei sicher feststehendem Konzeptionstermin (z. B. IVF/ICSI) – das (anamnestische) Gestationsalter korrigiert werden, wenn es mindestens 7 Tage vom sonografisch determinierten differiert 1 .
Bei nicht vorhandener SSL-Messung (z. B. bei spät diagnostizierter Schwangerschaft) kann evtl. auch der biparietale Durchmesser oder der transzerebellare Durchmesser zur Abschätzung des Gestationsalters herangezogen werden.
Eine Diskrepanz zwischen dem Gestationsalter berechnet nach der letzten Periode und nach Ultraschall kann ein erster Hinweis auf eine frühe Entwicklungsstörung sein. Eine weiterführende Abklärung und intensive Überwachung sind dann indiziert.
Neben dem Schätzgewicht ist der fetale Abdomenumfang der wichtigste Indikator für ein gestörtes fetales Wachstum. Zur Bestimmung des fetalen Schätzgewichts kann die Hadlock-Formel verwendet werden, die bei erhöhtem Risiko für eine FGR empfohlen wird 1 . Zu beachten gilt, dass Charakteristika der Eltern bei der Gewichtsschätzung berücksichtigt werden sollten.
Gemäß der Definition der FGR soll neben der aktuellen Gewichtsschätzung auch der Wachstumsverlauf berücksichtigt werden, um eine Wachstumsabflachung erkennen zu können, insbesondere da eine FGR nicht auf ein Gewicht unter der 10. Perzentile beschränkt ist.
Fruchtwasser
Die Fruchtwassermenge ist im Rahmen einer FGR häufig reduziert, da diese mit einer fetalen Oligurie einhergehen kann. Meist ist die Fruchtwassermenge jedoch nicht auffällig 1 , 14 . Zur Evaluation der Fruchtwassermenge können die „single deepest pocket“-Methode (SDP) oder die Messung des Amnion Fluid Index (AFI) angewandt werden 15 ; die SDP scheint zur Vorhersage eines Adverse Outcome besser geeignet 16 .
Erhärtet sich der V. a. auf eine SGA/FGR, soll eine weitere Abklärung zur Ursachenevaluation und Beurteilung des fetalen Zustandes erfolgen. Dies umfasst die dopplersonografische Beurteilung der uteroplazentaren Einheit (Aa. uterinae, A. umbilicalis) und ggf. weiterer fetaler Gefäße (A. cerebri media, Ductus venosus) und die sonografische Feindiagnostik. Zusätzlich kann eine differenzialdiagnostische genetische Abklärung einer Chromosomenanomalie und/oder einer Infektion des Fetus sinnvoll sein, um das weitere Management zu planen 1 .
Sonografische Feindiagnostik
Eine weiterführende, differenzierte Organdiagnostik (nach DEGUM-II-Kriterien) sollte zur differenzialdiagnostischen Abklärung einer möglichen FGR erfolgen ( Abb. 1 ) 1 . Zu beachten ist, dass strukturelle Auffälligkeiten des Fetus hinweisgebend auf genetisch-syndromale Erkrankungen sind, insbesondere bei frühen und multiplen Auffälligkeiten 17 . Zumindest dann sollte eine genetische Abklärung angeboten werden.
Doppler-Sonografie
Die dopplersonografische Untersuchung ist im Rahmen der Abklärung bei V. a. SGA/FGR zwingend erforderlich, einerseits zur differenzialdiagnostischen Unterscheidung einer SGA/FGR und andererseits zur Ursachenklärung einer FGR. Erhöhte Indizes in den Aa. uterinae und der A. umbilicalis weisen auf eine plazentare Störung i. S. einer Plazentainsuffizienz hin. Bei Diagnose einer FGR sollen weitere fetale Gefäße (A. cerebri media, Ductus venosus) zur Evaluation des fetalen Zustandes untersucht werden 1 .
Screening, Prädiktion und Prävention
Es gibt zurzeit noch keinen Screeningansatz mit guter Sensitivität und Spezifizität sowie negativem bzw. positivem Vorhersagewert 18 . Analog zur Ersttrimester-Risikoevaluation für Präeklampsie kann mit einer Kombination aus maternaler Anamnese, Doppler-Sonografie der Aa. uterinae, dem mittleren arteriellen Blutdruck und dem biochemischen Marker PAPP-A ein Screening auf eine fetale Wachstumsrestriktion durchgeführt werden 19 . Auch wenn die Detektionsrate nicht die Werte des Präeklampsiescreenings erreichen, kann damit ein Anteil der Schwangerschaften mit einem hohen Risiko für eine FGR detektiert werden und einer engmaschigen Überwachung zugeführt werden.
Im 2. Trimenon hat die dopplersonografische Untersuchung der Aa. uterinae in einem Niedrigrisikokollektiv nur einen limitierten Nutzen und im Risikokollektiv einen moderaten prädiktiven Nutzen für die frühzeitige Detektion einer FGR 20 . Daher wird die Durchführung einer Doppler-Sonografie der Aa. uterinae zum Screening auf FGR differenziert gesehen 21 . Ein auffälliger Befund sollte allerdings zu regelmäßigen sonografischen Wachstums- und Doppler-Kontrollen (Aa. uterinae, A. umbilicalis) führen.
Die Kombination der Doppler-Sonografie mit angiogenen Faktoren (z. B. sFlt-1/PlGF-Quotient) scheint die Vorhersage für eine FGR zu verbessern, ebenso wie die Kombination der fetalen Biometrie mit dem angiogenen Marker 22 , 23 , 24 . Allerdings sind auch hier bis zum weiten klinischen Einsatz noch weitere Studien notwendig.
Das antenatale Erkennen einer FGR ist essenziell, da neben dem Schwangerschaftsverlauf auch das neonatale Outcome positiv beeinflusst wird 2 , 25 . Nach wie vor werden international und auch in Deutschland trotz der engmaschigen Betreuung ein Großteil der FGR-Fälle pränatal nicht detektiert 1 , 25 .
Die Durchführung einer Fetometrie ist nach den Mutterschafts-Richtlinien zwischen der 18 + 0 und 21 + 6 Schwangerschaftswoche (SSW) und 28 + 0 und 31 + 6 SSW vorgesehen. Mit diesem Vorgehen ist die frühe FGR gut detektierbar, der Großteil der späten Wachstumsrestriktionen (ca. 70 – 80% der FGR) wird hiermit allerdings – insbesondere, wenn keine dopplersonografische Beurteilung oder spätere Biometrie erfolgt – nicht erkannt.
Um dies zu verbessern, sollte – gemäß der Definition der FGR – neben der aktuellen Gewichtsschätzung auch der Wachstumsverlauf berücksichtigt werden, um eine Wachstumsabflachung erkennen zu können, insbesondere da eine FGR nicht auf ein Gewicht unter der 10. Perzentile beschränkt ist. Bei Auffälligkeiten können dann weitere Untersuchungen, d. h. erneute Biometrie, dopplersonografische Beurteilung und ggf. Bestimmung der angiogenen Marker erfolgen 26 . Dies ist insbesondere von Bedeutung, da bei frühzeitiger/korrekter Erkennung einer FGR das perinatale Risiko reduziert werden kann 27 .
Eine (zusätzliche) späte Biometrie bei ≥ 36 SSW verbessert die Detektionsrate einer FGR um das 3-Fache 28 , 29 . Kombiniert mit der Beurteilung des fetalen Wachstumsverlauf kann eine Subgruppe mit hohem perinatalem Risiko detektiert werden 28 , 30 . Dopplersonografische Auffälligkeiten (maternal, fetoplazentar oder fetal) sind mit einem ungünstigeren perinatalen Outcome assoziiert 27 , 31 , 32 . 15 – 20% der späten FGR zeigen – bei unauffälligem Blutfluss in der Nabelschnur – Auffälligkeiten der zerebralen Perfusion; durch die Bestimmung der zerebroplazentaren Ratio (CPR) können Feten (mit und ohne Wachstumsproblematik) mit einem erhöhten Risiko für ein ungünstiges perinatales Outcome besser detektiert werden 33 , 34 , 35 . Die Kombination Biometrie, uteriner Doppler und CPR im 3. Trimester kann den Großteil der Feten mit hohem Risiko für einen intrauterinen Fruchttod (IUFT) detektieren 31 , scheint aber im unselektierten Kollektiv nur geringen Nutzen zur Detektion einer SGA/FGR zu haben 36 . Auch die Kombination der fetalen Biometrie mit der Bestimmung angiogener Faktoren (insbesondere des sFlt-1/PlGF-Quotienten) scheint für die Detektion einer FGR nützlich zu sein 23 , 37 , 38 .
Im Gegensatz zur Präeklampsie scheint die Gabe von niedrig dosiertem Aspirin zur Prävention einer FGR nur mäßig erfolgreich zu sein (wobei allerdings die Studie nicht für die Prävention einer FGR statistisch ausreichende Power hatte) 39 , auch hier sind weitere Studien notwendig. Die Ergebnisse der deutschen Multicenterstudie zum Nutzen des NO-Donors PETN bei Frauen mit pathologischem Doppler der Aa. uterinae im 2. Trimenon sind in Kürze zu erwarten 40 .
Betreuung SGA/FGR
Das Management einer FGR ist für alle Beteiligten eine Herausforderung: Eine fetale Hypoxämie soll frühzeitig erkannt werden, um einen irreversiblen Schaden oder einen intrauterinen Fruchttod zu vermeiden. Andererseits soll eine Schwangerschaft nicht zu früh beendet werden, um die Folgen einer Frühgeburtlichkeit so gering wie möglich zu halten.
In alle Entscheidungen müssen die Eltern einbezogen und die Konsequenzen der verschiedenen Optionen dargelegt werden. Insbesondere das erhöhte Risiko eines intrauterinen Fruchttodes bei zuwartendem Vorgehen muss dem erhöhten Mortalitäts- und Morbiditätsrisiko der Frühgeburtlichkeit bei Entbindung gegenübergestellt werden. Eine enge prä- und perinatale Zusammenarbeit mit den Neonatologen ist daher erforderlich, um den Eltern ausreichend Informationen zur Verfügung zu stellen.
Zu beachten ist, dass eine frühe FGR und eine späte FGR unterschiedlich beurteilt werden sollen. Eine zunehmende Verschlechterung spiegelt sich bei einer frühen FGR in Auffälligkeiten venöser Doppler-Parameter wider, bei einer späten FGR vor allem in der zerebralen Doppler-Sonografie ( Tab. 2 ) 1 , 41 .
Tab. 2 Frühe/späte FGR (nach 42 ).
Eine einzelne Überwachungsmethode kann das Outcome einer FGR nicht valide vorhersagen, es wird eine Kombination verschiedener Verfahren empfohlen. Insbesondere Sonografie und vor allem Doppler-Sonografie sind in der Überwachung einer FGR essenziell, um das perinatale Outcome zu verbessern.
Die Betreuung von Schwangeren mit SGA- oder FGR-Feten erfolgt befundadaptiert mit einer Kombination verschiedener Untersuchungsmethoden, die in Abb. 2 zusammengefasst ist 1 . Kontrollen des fetalen Wachstums und der Fruchtwassermenge, fetaler arterieller und venöser Gefäße gehören zu obligaten Maßnahmen; CTG-/Computer-CTG-Kontrollen liefern darüber hinaus wichtige Informationen über den fetalen Zustand 1 , 42 .

Betreuungsschema bei fetaler Wachstumsrestriktion (Daten aus 1 ).
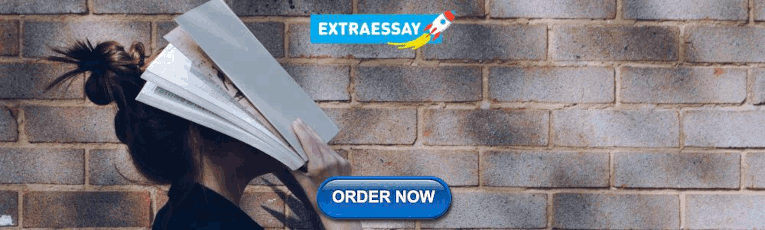
Biometriekontrollen
Serielle sonografische Kontrollen des fetalen Wachstums sind unabdingbar bei der Betreuung von SGA/FGR-Feten. Es gilt jedoch zu beachten, dass – auch aufgrund der Limitationen der sonografischen Gewichtsschätzung – die Untersuchungsintervalle nicht zu kurz sein sollten: der Abstand zwischen den Untersuchungen sollte mindestens 2, besser 3 Wochen betragen 1 , 43 .
- A. umbilicalis: Die dopplersonografische Untersuchung der A. umbilicalis erlaubt die Detektion von hämodynamischen Veränderungen im Verlauf. Normale Widerstandsindizes weisen auch bei FGR auf ein geringes Risiko für ein ungünstiges perinatales Outcome und eine geringe perinatale Mortalität hin 9 . Die Prognose verschlechtert sich in Abhängigkeit vom Schweregrad der Widerstandserhöhung 41 . Bei einem sogenannten ARED-Fluss (absent/reversed enddiastolic flow) sind ca. 70% der plazentaren Gefäße verschlossen 44 , was in einem 5-fach höheren Risiko für perinatale Mortalität bei „reversed enddiastolic flow“ resultiert. Basierend auf Studien zur Progression der Doppler-Verschlechterungen 45 werden die in Abb. 2 genannten Untersuchungsintervalle empfohlen. Bei einem unauffälligen Widerstand in der A. umbilicalis scheinen 2-wöchige Intervalle ausreichend zu sein 46 .
- Ductus venosus: Für die Betreuung der frühen FGR ist der Ductus venosus ein entscheidendes Gefäß 41 , im Management der frühen FGR soll die Evaluation des Ductus venosus zur Beurteilung verwendet werden 1 . Veränderungen der venösen Strombahn bis hin zur Flussumkehr treten gewöhnlich später als Veränderungen im arteriellen Gefäßsystem auf 41 . Pathophysiologisch resultiert eine zunehmende kardiale Funktionseinschränkung in einem abnehmenden diastolischen Blutfluss bzw. einer Zunahme der Pulsatilität im Ductus venosus bis zum Verlust der positiven a-Welle; eine fehlende a-Welle oder ein reverse flow ist ein Hinweis für eine kardiovaskuläre Instabilität und kann ein Zeichen für eine drohende oder auch bereits bestehende Azidämie sein 47 , 48 , 49 , das Risiko für einen intrauterinen Fruchttod verdoppelt sich täglich 45 , 47 .
- A. cerebri media: Mit der Untersuchung der A. cerebri media (ACM) kann eine zunehmende Hypoxämie detektiert werden, da es im Rahmen der zunehmenden fetalen Kompromittierung zu einer Umverteilung des Blutes („brain-sparing effect“) kommt 41 . Der Widerstand in der ACM nimmt ab und gilt ab einem Pulsatilitätsindex (PI) < 5. Perzentile als pathologisch 1 . Bei einer frühen FGR ist die prädiktive Aussage der ACM hinsichtlich der Vorhersage eines ungünstigen perinatalen Outcomes limitiert 50 , 51 , 52 , Bedeutung gewinnt die Untersuchung der ACM, vorzugsweise in Kombination mit der A. umbilicalis als sogenannte zerebroplazentare Ratio (CPR), bei der Betreuung der späten FGR. Mit der CPR ist einerseits eine exaktere Diagnose der späten FGR möglich 42 , andererseits zeigen verschiedene Studien einen Nutzen zur Vorhersage eines ungünstigen perinatalen Outcomes bei pathologischer CPR, d. h. Werten der Ratio zwischen A. umbilicalis und A. cerebri media (< 5. Perzentile oder < 1) 53 , 54 , 55 , 56 .
(Computer-)Kardiotokografie-Kontrollen (cCTG)
Die Kardiotokografie (CTG) sollte gemäß den Mutterschaftsrichtlinien im Rahmen der Schwangerenvorsorge bei Verdacht auf eine Plazentainsuffizienz durchgeführt werden 1 . Sie detektiert jedoch eher akute als chronische Verläufe 41 und sollte daher nie als alleinige Überwachungsmethode bei der Betreuung einer FGR eingesetzt werden 1 .
Um die Limitation einer CTG-Untersuchung (subjektive Beurteilung) zu minimieren, kann eine computerbasierte Analyse des CTGs verwendet werden. Die höhere Objektivität der Bewertung sowie die Möglichkeit, die Kurzzeitvariabilität (= short-term Variation, STV) zu analysieren, sind wesentliche Vorteile dieser Methode 57 . Wie bei unkomplizierten Schwangerschaften steigt auch bei einer FGR die STV mit dem Gestationsalter an, es werden jedoch generell niedrigere STV aufgezeichnet 58 . Eine STV von 4,5 ms schließt eine fetale Azidämie aus (NPV 100%) 59 , abnehmende STV-Werte sind mit früherer Entbindung, niedrigerem Geburtsgewicht, niedrigerem pH-Wert der Nabelschnurarterie, schlechterem Säure-Basen-Status und schlechteren neonatalen Outcome assoziiert 60 .
Durch die Messung der STV und die Beobachtung des zeitlichen Verlaufs können somit ggf. subtile Veränderungen entdeckt werden, welche zum besseren Timing der Entbindung hilfreich sein können 61 , was den Einsatz der Methode bei der Betreuung einer FGR unterstreicht; dennoch muss beachtet werden, dass CTG-Veränderungen sich bei FGR erst relativ spät zeigen 41 , 61 und dass auch die Kurzzeitvariabilität medikamentösen Einflüssen (z. B. bei antenataler Kortikoidsteroidgabe) unterworfen ist und daher bei der Interpretation berücksichtigt werden muss 1 , 62 , 63 .
Sonstige Maßnahmen
Ambulante/stationäre betreuung.
Schwangere mit einer FGR können generell ambulant betreut werden, da es keine evidenzbasierten Daten gibt, auf deren Grundlage Indikationen für eine stationäre Überwachung festgelegt werden können. Bei zunehmendem Schweregrad bzw. kindlicher Beeinträchtigung kann jedoch eine stationäre Überwachung hilfreich sein, wenn engmaschige Untersuchungsintervalle notwendig werden. Zu beachten gilt auch die Assoziation einer frühen FGR mit einer Präeklampsie. Die Entscheidung für eine ambulante oder stationäre Betreuung sollte individuell gefällt werden 1 .
Antenatale Kortikoidsteroidgabe
Eine Herausforderung in der Geburtshilfe (und somit auch bei der Betreuung einer FGR) stellt das Abschätzen des Zeitpunkts der voraussichtlich erforderlichen Entbindung in der Frühgeburtssituation dar. Wenn von einer Entbindung innerhalb der nächsten 7 Tage auszugehen ist, sollten Kortikosteroide verabreicht werden 1 , 64 . Die Vorteile der antenatalen Kortikosteroide sind auch bei wachstumsrestringierten Feten vorhanden. Zu beachten ist allerdings, dass es unter der Kortikosteroidgabe zu einer vorübergehenden Reduktion der Herzfrequenzvariation und einer Verringerung der Körper- und Atembewegungen des Fetus kommen kann; diese Veränderungen normalisieren sich innerhalb von 72 Stunden. 1 .
Neben dem o. g. „timing“ der Steroidgabe gilt generell, dass eine unkritische Applikation von Steroiden bei allen FGR nicht erfolgen soll, da Kortikosteroide – neben den unbestrittenen positiven Effekten – auch verschiedenste negative Auswirkungen (u. a. reduziertes Wachstum, verzögerte neurologische Entwicklung, Stress, Hypertonie) aufweisen. Zudem weisen FGR-Feten, insbesondere bei zunehmender Hypoxämie, bereits hohe Cortisolspiegel auf, und nach 30 SSW entwickeln FGR-Feten wesentlich seltener ein RDS als eutrophe Feten. Ausreichenden Daten zum Effekt der Steroide auf das Langzeit-Outcome bei FGR-Feten > 30 SSW liegen nicht vor.
Ziel sollte daher sein, eine „Lungenreifeinduktion“ und erst recht eine wiederholte antenatale Steroidgabe bei Frauen wenn irgend möglich zu vermeiden, die letzten Endes keine Frühgeburt erleiden 65 .
Magnesium zur Neuroprotektion
Die antenatale Gabe von Magnesium i. v. scheint einen neuroprotektiven Effekt zu haben und kann somit zur Reduktion des Risikos neurologischer Schäden appliziert werden. Die FGR ist mit einem erhöhten Frühgeburtsrisiko assoziiert, weshalb (inter-)nationale Fachgesellschaften die Gabe von Magnesiumsulfat zur Neuroprotektion vor 32 + 0 SSW bei unmittelbar drohender Geburt empfehlen 1 , 64 .
Geburt/Entbindung bei SGA/FGR
Entbindungsort.
Bei Vorliegen einer FGR soll die Entbindung in einem Perinatalzentrum mit neonataler Intensivstation und erfahrenem Team erfolgen, um eine sofortige und kontinuierliche Betreuung zu gewährleisten 1 .
Entbindungszeitpunkt
Bei der Planung des Zeitpunkts der Entbindung sollen die Risiken der Frühgeburtlichkeit mit denen des intrauterinen Verbleibs und/oder den maternalen Risiken abgewogen werden. Maternale Entbindungskriterien gelten unabhängig vom Gestationsalter und Ausmaß der FGR.
Aus fetaler Sicht orientiert sich das Timing des Entbindungszeitpunkts neben dem Gestationsalter vor allem an den Befunden der Doppler-Sonografie ( Abb. 2 ). Wie bereits erwähnt, zeigt sich bei einer frühen FGR eine gravierende Verschlechterung insbesondere in Pathologien venöser Gefäße (Ductus venosus), bei der späten FGR in Pathologien der zerebralen Gefäße (ACM, CPR). Dies spiegelt sich in den Empfehlungen (inter-)nationaler Leitlinien und Experten wider 1 , 42 , 66 , 67 .
Anmerkung: Die im Folgenden genannten Cut-off-Werte (cCTG und Ductus venosus) sind – ebenso wie Dezelerationen – Spätzeichen der fetalen Zustandsverschlechterung. In einigen dieser Fälle ist bereits eine myokardiale Dysfunktion und möglicherweise auch Hypotension eingetreten, die fetalen Anpassungsmechanismen erfüllen ihre Schutzfunktion bereits nicht mehr. Dies bedeutet, dass mit zunehmendem Gestationsalter die Bedeutung dieser Parameter geringer wird.
- cCTG: Bei auftretenden CTG-Pathologien (rezidivierende, spontane und therapieresistente Dezelerationen zu jedem Zeitpunkt) soll eine Entbindung in Erwägung gezogen werden 1 , 61 . Zwischen der 26 + 0 – 28 + 6 SSW soll bei einer STV < 2,6 ms und zwischen 29 + 0 – 32 + 0 SSW bei < 3,0 ms die Entbindung in Erwägung gezogen werden ( Abb. 2 ) 1 , 61 .
- Ductus venosus (frühe FGR): Nach derzeitigem Kenntnisstand können pathologische Befunde des Ductus venosus eine Entbindungsindikation darstellen, wenn der Fetus lebensfähig ist und antenatale Kortikosteroide appliziert wurden 1 . Abhängig vom Gestationsalter und ggf. Zusatzbefunden ist mit der Schwangeren bei erhöhtem Widerstand (> 95. Perzentile – einhergehend mit einer erniedrigten a-Welle) eine Entbindung zu diskutieren; bei fehlender oder reverser a-Welle ist die Entbindung indiziert 1 , 41 , 42 , 61 , 66 .
Die „optimale“ Methode zur Überwachung einer frühen FGR stellt nach derzeitigem Kenntnisstand die Kombination von computerisiertem CTG und Ductus venosus dar 68 . Bei unauffälliger Doppler-Sonografie des Ductus venosus und fehlenden cCTG-Auffälligkeiten kann es dennoch sinnvoll sein, die Schwangerschaft früher zu beenden.
- A. umbilicalis: Bei Absent oder Reversed enddiastolic flow (AREDF) der A. umbilicalis kann die Prognose schlecht sein 1 , 41 , 69 , allerdings ist auch die frühgeburtsassoziierte Morbidität und Mortalität vor 32 + 0 SSW relativ hoch 70 und ein intrauteriner Verbleib des Fetus bringt deutliche Verbesserungen mit sich 71 . Dies berücksichtigend, empfiehlt die aktuelle Leitlinie bei ARED-Flow: Bei einem REDF kann daher ab 30 + 0 SSW eine Entbindung in Erwägung gezogen werden und sollte mit 32 + 0 SSW erfolgen. Das Mortalitätsrisiko ist bei einem Absent enddiastolic Flow (AEDF) geringer, jedoch sollte ab 34 + 0 SSW die Schwangerschaft beendet werden 1 . Ein Zuwarten bis zu diesen Schwangerschaftswochen ist – bei ansonst unkompliziertem Verlauf – möglich und zeigt keinen signifikanten Unterschied hinsichtlich Mortalität und Morbidität nach 2 Jahren 72 . Ein erhöhter Widerstand in der A. umbilicalis (PI > 95. Perzentile) ist ebenso mit einem erhöhten perinatalen Risiko für Mortalität und Morbidität assoziiert, allerdings mit geringem prädiktivem Wert. Bei erhöhtem PI > 95. Perzentile wird die Entbindung daher ab 37 + 0 SSW empfohlen 1 .
- A. cerebri media/zerebroplazentare Ratio: In der Frühgeburtssituation (< 37 + 0 SSW) ist der prädiktive Wert der ACM nur von begrenztem Nutzen, um eine Azidämie oder ein schlechtes perinatales Outcome vorherzusagen, sie sollte daher nicht zur Entscheidung bez. des Entbindungszeitpunkts in dieser Phase herangezogen werden 1 . Ab 37 + 0 SSW sollte bei einer erniedrigten Widerstand in der ACM (PI < 5. Perzentile) die Entbindung erwogen werden 1 . Die zerebroplazentare Ratio (CPR) wird derzeit noch kontrovers diskutiert, da vor allem genaue Grenzwerte nicht eindeutig evaluiert sind. Da in einigen Arbeiten eine pathologisch erniedrigte CPR ein Prädiktor für ein schlechtes perinatales Outcome ist, kann ab 37 + 0 SSW die Entbindung angestrebt werden 1 .
Da ein isolierter SGA-Fetus, d. h. fetales Wachstum < 10. Perzentile, unauffälliger Doppler-Befund und keine Zusatzrisiken, trotz im Allgemeinen günstigem Outcome ein erhöhtes Risiko für einen intrauterinen Fruchttod aufweist, kann auch bei SGA-Feten eine frühere Entbindung ab 38 + 0 SSW in Erwägung gezogen werden. Eine Terminüberschreitung soll bei einem isolierten SGA-Fetus vermieden werden 1 , da letztlich eine sichere Unterscheidung zwischen SGA und FGR mit den derzeitigen Methoden der fetalen Überwachung (Biometrie, utero-umbiliko-fetaler Doppler, biophysikalisches Profil, Non-Stress-Test) nicht in allen Fällen gelingt und es in Terminnähe zunehmend zu einer relativen uteroplazentaren Insuffizienz kommt.
Ein isolierter Wachstumsstillstand ist – bei unauffälligem Doppler und cCTG – kein unabhängiger Faktor für eine sofortige Beendigung der Schwangerschaft. In diesen Konstellationen sollte stets das Gestationsalter berücksichtigt und das Messintervall überprüft werden, um den methodischen Schätzfehler der sonografischen Gewichtsschätzung zu minimieren 1 .
Geburtsmodus
Verschiedene Faktoren wie das Vorliegen pathologischer Befunde (Doppler, cCTG) oder sonstige fetale oder maternale Besonderheiten oder Komplikationen müssen neben Gestationsalter, Parität und Zervixreife für die Wahl des Geburtsmodus berücksichtigt und es muss individuell eine Entscheidung getroffen werden 1 :
Bei einer FGR mit unauffälligen Doppler-Befunden oder erhöhter Pulsatilität in der A. umbilicalis (> 95. Perzentile) – nicht bei ARED-Flow – kann eine Geburtseinleitung durchgeführt und eine Vaginalgeburt angestrebt werden. Allerdings muss das höhere Komplikationsrisiko beachtet werden und intrapartal eine kontinuierliche Überwachung erfolgen 1 .
Bei pathologischem Doppler-Befund im Sinne eines erhöhten Widerstandes in der A. umbilicalis (nicht ARED-Flow) oder bei später FGR bei pathologischem Befund der ACM/CPR ist eine Geburtseinleitung und eine Vaginalgeburt möglich, wobei eine kontinuierliche Überwachung sub partu obligat erfolgen muss 1 .
Bei früher FGR wird bei pathologischen cCTG, auffälligem Ductus venosus und/oder insbesondere ARED-Flow, schon alleine wegen der erhöhten kindlichen Kompromittierung, die diese Situationen anzeigen, meist ein Kaiserschnitt durchgeführt und empfohlen. Auch in sehr frühen Schwangerschaftswochen muss bei indizierter Beendigung der Schwangerschaft aufgrund der fehlenden Möglichkeit einer sinnvollen Geburtseinleitung ein Kaiserschnitt durchgeführt werden 1 .
REVIEW article
Fetal growth restriction and stillbirth: biomarkers for identifying at risk fetuses.
- 1 Fetal Physiology and Neuroscience Group, Department of Physiology, The University of Auckland, Auckland, New Zealand
- 2 Department of Obstetrics and Gynaecology, The University of Auckland, Auckland, New Zealand
- 3 Auckland Biomedical Engineering Institute, The University of Auckland, Auckland, New Zealand
Fetal growth restriction (FGR) is a major cause of stillbirth, prematurity and impaired neurodevelopment. Its etiology is multifactorial, but many cases are related to impaired placental development and dysfunction, with reduced nutrient and oxygen supply. The fetus has a remarkable ability to respond to hypoxic challenges and mounts protective adaptations to match growth to reduced nutrient availability. However, with progressive placental dysfunction, chronic hypoxia may progress to a level where fetus can no longer adapt, or there may be superimposed acute hypoxic events. Improving detection and effective monitoring of progression is critical for the management of complicated pregnancies to balance the risk of worsening fetal oxygen deprivation in utero , against the consequences of iatrogenic preterm birth. Current surveillance modalities include frequent fetal Doppler ultrasound, and fetal heart rate monitoring. However, nearly half of FGR cases are not detected in utero , and conventional surveillance does not prevent a high proportion of stillbirths. We review diagnostic challenges and limitations in current screening and monitoring practices and discuss potential ways to better identify FGR, and, critically, to identify the “tipping point” when a chronically hypoxic fetus is at risk of progressive acidosis and stillbirth.
Introduction
Fetal growth restriction (FGR) is defined as a fetus that fails to reach its biologically determined growth potential ( Blencowe et al., 2019 ; Biks et al., 2021 ). The global prevalence is suggested to be around 20.5% ( Blencowe et al., 2019 ). The incidence is reported to be higher in low- and middle-income countries; although accurate data are lacking ( Blencowe et al., 2019 ; Biks et al., 2021 ). FGR is strongly associated with adverse pregnancy outcomes including stillbirth, preterm birth, increased neonatal mortality, increased risk for adult diseases, and neurodevelopmental disability ( Malhotra et al., 2019 ; Fung and Zinkhan, 2021 ; Dudink et al., 2022 ). In principle, accurately identifying and managing FGR cases should be central to reducing mortality and morbidity. In practice, currently more than 50% of cases of FGR are undetected even in high income countries ( McCowan et al., 2018 ; Malhotra et al., 2019 ), and more than 70% of babies with FGR who die antepartum are not diagnosed ( Pacora et al., 2021 ). Further, even when FGR is correctly identified, there are only limited tools to monitor the severity of fetal oxygen deprivation, and thereby attempt to balance the risks of stillbirth or impaired development against the risks of premature delivery ( Damhuis et al., 2021 ; Lausman and Kingdom, 2021 ). In this review, we will discuss the diagnostic challenges for FGR, the limitations of available monitoring techniques, and potential biomarkers that may help to improve detection and management of FGR.
Small for gestational age vs. fetal growth restriction: Definitions
One of the key difficulties in developing biomarkers to better identify FGR is distinguishing those who are truly growth restricted due to pathological circumstances (e.g. oxygen and nutrient deprivation) from those who are small for gestational age (SGA), but have normal placental gas exchange or are healthy ( Khalil et al., 2015 ; Damhuis et al., 2021 ; Lees et al., 2022 ). This is a major problem for research, since if a biomarker discovery cohort included a mixed group of infants with SGA, only some of whom had FGR, the inconsistency in definition would confound any association.
SGA fetuses are typically defined by an estimated birth weight below the 10th percentile or less than two standard deviations below the mean for their age, sex and parity. There is growing evidence that birth weight standards customized to specific populations, and incorporating factors such as ethnicity and maternal height and body mass index, can improve identification of FGR at preterm gestational ages ( Anderson et al., 2016 ; Gardosi et al., 2018 ; Damhuis et al., 2021 ; Lees et al., 2022 ). SGA and FGR are not synonymous; in practice, FGR is an overlapping subset of SGA ( Gordijn et al., 2016 ; Damhuis et al., 2021 ; Deter et al., 2021 ). Most infants with early-onset FGR are SGA, though around 40% of babies <10th percentile are constitutionally small and healthy ( Khalil et al., 2015 ).
Conversely, late-onset FGR with placental insufficiency may lead to restricted fetal growth compared to their growth potential, but with a birth weight in the normal range (i.e. be AGA) ( Gordijn et al., 2016 ; Damhuis et al., 2021 ; Deter et al., 2021 ). Up to 70% of deaths before birth occur in fetuses who were considered to be AGA ( Pacora et al., 2021 ). Heterogeneity of growth throughout gestation further complicates accurate diagnosis, highlighting the value of individualized longitudinal assessments ( Deter et al., 2021 ).
These data tell us that although fetuses whose weight is < 3rd percentile have significantly higher risks of adverse events, fetal size alone cannot be sufficient to accurately predict FGR and its associated risks ( Unterscheider et al., 2013 ). It is now recognized that accurately identifying FGR and thus risk requires a broader suite of measures as proposed by the Delphi procedure consensus criteria ( Gordijn et al., 2016 ; Beune et al., 2018 ). In addition to redefining estimated fetal weight to better stratify risk for very small (weight <3rd percentile) and small (>3rd to <10th percentile) babies and assessing maternal comorbidities, further assessment variables include placental, umbilical and fetal blood flow, fetal heart rate analysis, gestational age, assessment of the fetal biophysical profile and longitudinal growth trajectory and diagnostic and/or prognostic biochemistry ( Gordijn et al., 2016 ; Beune et al., 2018 ). Numerous studies are now assessing and validating these measurements, adding to the data obtained from other recent assessment trials such as the Growth Restriction Intervention Study (GRIT) and The Trial of Umbilical and Fetal Flow in Europe (TRUFFLE) ( Khalil et al., 2019 ; Ganzevoort et al., 2020 ; Lausman and Kingdom, 2021 ).
It is important to appreciate that FGR is a biological continuum. The timing of FGR is an important variable. Around 20–30% of FGR cases are early-onset (onset <32 weeks gestation). These fetuses have much greater risk of mortality and morbidity ( Figueras and Gratacós, 2014 ; Mifsud and Sebire, 2014 ; Dall’Asta et al., 2017 ; Baschat, 2018 ; Lees et al., 2022 ). Late-FGR (≥32 weeks) is still associated with risk of adverse perinatal events and outcomes, including late preterm birth, sudden fetal deterioration and hypoxia and stillbirth ( Baschat, 2018 ; Figueras et al., 2018 ).
The etiology of FGR is multifactorial, and can include impaired placental development and function, pre-eclampsia and maternal hypertension, and poor maternal cardiovascular adaptation to pregnancy, multiple gestation, maternal diabetes, maternal environment (e.g. high altitude, asthma, and stress) and lifestyle factors such as smoking, drug-use, malnutrition, and placental and fetal abnormalities (genetic or otherwise) ( Sharma et al., 2016 ; Burton and Jauniaux, 2018 ; Damhuis et al., 2021 ; Nowakowska et al., 2021 ). Infections such as cytomegalovirus, syphilis and hepatitis C can also be associated with FGR ( Longo et al., 2014 ). SARS-Cov2 infection may cause FGR secondary to the formation of placental lesions and placental insufficiency ( Dubucs et al., 2022 ).
Ultimately, the most common underlying pathogenesis involves impaired placental development and function, leading to reduced supply of nutrients and oxygen ( Mifsud and Sebire, 2014 ; Burton and Jauniaux, 2018 ; Zur et al., 2020 ). Maternal vascular malperfusion is the most common placental cause linked with FGR. It can arise from a variety of placental abnormalities, which can be interconnected, from placental hypoplasia to infarcts and lesions ( Zur et al., 2020 ). Failure of extravillous cytotrophoblast invasion leading to poor placental structure and inadequate remodeling of spiral arteries (the maternal arteries that directly supply the placenta), for example, impairs perfusion ( Burton and Jauniaux, 2018 ), giving rise to altered vascular resistance, intra-placental vascular lesions and reduced surface area for maternal-fetal exchange ( Mifsud and Sebire, 2014 ). Further, impaired placentation is associated with secondary factors that alter placental perfusion such as maternal hypertension and pre-eclampsia ( Zur et al., 2020 ). While small, dysfunctional placentae are at the root of early-onset FGR and more severe FGR, impaired placental structure and function also underpins late-onset FGR. The difference is a matter of degree rather than different causal mechanisms per se ( Figueras et al., 2018 ). Late-onset FGR may be associated with an increased incidence of fibrosis and reduced vascularity ( Kovo et al., 2010 ; Mifsud and Sebire, 2014 ; Figueras et al., 2018 ).
Fetal adaptation to growth restriction–vulnerability to further hypoxia?
The fetus can detect and respond to hypoxic challenges to its homeostasis from very early in gestation (0.3 gestation, as seen in fetal sheep), and makes adjustments to match growth with energy availability if the hypoxia persists and the fetus is able to adapt appropriately ( Kiserud et al., 2001 ; Giussani, 2016 ; Bennet, 2017 ; Lear et al., 2018 ).
Early-onset FGR is usually characterized by symmetrical growth, where reduced growth of the head and body are in proportion. By contrast, later-onset FGR is typically characterized by asymmetrical growth, where the abdominal circumference is reduced <10th percentile, while other measurements (especially head circumference) are relatively preserved and may be within normal limits ( Figueras et al., 2018 ). Asymmetrical growth is thus associated with so-called “brain sparing,” with redistribution of blood flow away from the periphery to central beds to support organs such as the brain ( Kiserud et al., 2001 ; Giussani, 2016 ; Bennet, 2017 ; Lear et al., 2018 ). The word “sparing” should not, however, be taken to mean normal brain growth in all cases, given that brain injury and impaired brain development are observed in even fetuses with asymmetrical FGR ( Miller et al., 2016 ; Dudink et al., 2022 ). Indeed, brain sparing is not associated with improved executive function and behavioral outcomes or cognitive function in school-age children ( Benítez-Marín et al., 2021 ). Neurological sequelae undoubtedly reflect that FGR is a continuum, and the specific outcomes must reflect the timing of placental insufficiency (i.e. stage of neural development) as well as severity of the challenge ( Miller et al., 2016 ; Malhotra et al., 2019 ; Dudink et al., 2022 ).
Insights from animal models
Critically, pre-clinical studies in a variety of species demonstrate that FGR is also associated with effects on other organs such as the kidney, with reduced nephron endowment and associated reduced filtration capacity ( Mitchell et al., 2004 ), and heart, with reduced cardiac myocyte endowment, impaired maturation, remodeling of cardiac vasculature and a switch in energy substrate use ( Louey et al., 2007 ; Wang et al., 2013 ; Botting et al., 2018 ; Masoumy et al., 2018 ; Maréchal et al., 2021 ; Drake et al., 2022 ). Altered renal and cardiac development are strongly linked with later-life risks for cardiovascular disease ( Masoumy et al., 2018 ; Fung and Zinkhan, 2021 ). However, altered cardiac maturation and energy management may also affect fetal survival during further episodes of hypoxia. For example, a recent study evaluating cardiac metabolism in growth retarded fetal sheep using two imaging modalities, two-photon microscopy and phase-contrast magnetic resonance imaging, found evidence that the FGR heart relies heavily on glycolysis for ATP production, consistent with an impaired ability to tolerate further hypoxia ( Dimasi et al., 2021 ). We have previously shown that fetal weight was strongly associated with impaired tolerance to severe hypoxia in preterm fetal sheep, particularly in males, and that the pattern of impaired failure of tolerance to hypoxia was sex dependent ( Bennet et al., 2007 ). Others report that FGR reduces cardiac myocyte endowment in a sex-specific manner ( Botting et al., 2018 ) and alters cardiac energy management ( Maréchal et al., 2021 ).
Hypoxia secondary to placental dysfunction is a key feature of stillbirth, both as a direct feature of placental dysfunction and because it is associated with exacerbation of fetal compromise during secondary insults. Thus, it is vital to understand whether fetuses with FGR have compromised ability to respond to further hypoxic challenges ( Pacora et al., 2019 ). The fetal chemoreflex and other defensive adaptations to hypoxia and more severe insults such as asphyxia are well described (for reviews see ( Giussani, 2016 ; Lear et al., 2016 ; Bennet, 2017 )). In chronically instrumented fetal sheep, the pattern of fetal adaptation to severe hypoxic challenge is similar at all gestational ages, with similar stages of compensation before final decompensation, but that younger fetuses are able to survive severe hypoxia for much longer than older fetuses, consistent with their known anaerobic tolerance and high levels of cardiac glycogen ( Dawes et al., 1959 ; Shelley, 1964 ; Bennet, 2017 ). Unfortunately, surviving and thriving are very different, and to date, there is surprisingly limited data on the responses of the FGR fetus to superimposed episodes of acute hypoxia.
Acute hypoxia superimposed on chronic hypoxia may contribute to some antenatal and intrapartum fetal deaths ( Pacora et al., 2019 ). For others, there may simply come a point when placental reserve fails and chronic hypoxia transitions to effectively represent acute severe hypoxia. Under such conditions the fetus must make further defensive responses, which include further redistribution of blood flow to vital organs, and conservation of energy through reduced body and breathing movements and other fetal activity ( Pagani et al., 2014 ; Binder et al., 2018 ). Reduced fetal movements are strongly associated with fetal death ( Heazell et al., 2018a ; Ter Kuile et al., 2021 ). Further, abrupt fetal movements have been observed before stillbirth ( Heazell et al., 2018b ; Thompson et al., 2021 ). It has been speculated that this may be related to fetal seizures ( Heazell et al., 2018b ; Whitehead et al., 2020 ). Alternatively though, in fetal sheep, abrupt movements are seen shortly after the onset of an acute asphyxial insult ( Bennet, 2017 ).
Studies in fetal sheep models have examined the impact of chronic hypoxia on the fetus’ ability to respond to acute insults, although many questions remain. In healthy near-term fetal sheep, brief umbilical cord occlusions repeated at a frequency that is consistent with early labor (1 min of occlusion every 5 min) can be fully compensated for, with no hypotension and only mild acidosis ( Bennet et al., 2005 ). Near-term fetal sheep with pre-existing hypoxia of unknown origin and duration exposed to the same challenge show enhanced initial chemoreflex response to hypoxia ( Bennet et al., 2005 ) and earlier centralization of blood flow ( Wibbens et al., 2007 ). However, these early defense responses are not maintained; fetuses with pre-existing hypoxia become metabolically acidotic and hypotensive during repeated umbilical cord occlusion compared with healthy fetuses, and experience more severe decompensation ( Westgate et al., 2005 ; Pulgar et al., 2006 ; Wassink et al., 2013 ; Amaya et al., 2016 ). These data suggest that autonomic responses to hypoxia are intact in FGR, and in some respects enhanced, but anaerobic reserves such as cardiac glycogen are insufficient to maintain cardiovascular adaptation.
There is some evidence of altered responses from preclinical studies in induced FGR. In a classic study, embolization of the placental circulation of late gestation (0.75 gestation) fetal sheep for ∼2 weeks induced moderate hypoxia without acidosis, resulting in a 31% reduction in fetal growth, and redistribution of combined ventricular output with an increased brain-liver ratio (asymmetrical growth) ( Block et al., 1989 ). The fetuses were then challenged with a short period of isocapnic hypoxia induced by reduction of the maternal FiO 2 to ∼10%. The FGR fetuses mounted a greater circulatory defense response than control fetuses, but without a change in umbilical blood flow. The authors queried if this could be sustained, and if better early compensation would be followed by quicker decompensation. A study of only 9 days embolization producing a 20% reduction in fetal growth and similar hypoxia, showed no redistribution of blood flow prior to the acute hypoxic challenge (symmetrical growth), and no differences in the circulatory response to acute hypoxia between FGR and control groups ( Block et al., 1990 ). The authors concluded that under these circumstances the FGR fetuses were able to mount a comparable cardiovascular defense to hypoxia, and would do so until the placental reserve was completely depleted.
An alternative approach is to induce growth restricted pregnancy by removal of placental sites pre-implantation (carunculectomy) ( Robinson et al., 1983 ). In this study, fetal weight was highly variable, ranging from no change to a 60% reduction compared with controls, but all fetuses were hypoxic without acidosis. In response to superimposed isocapnic hypoxia, FGR fetuses initially responded with acute bradycardia, similarly to controls, but interestingly, heart rate returned to baseline within a short period. This likely reflects higher circulating levels of catecholamines as reported in this model ( Llanos et al., 1980 ; Walker et al., 1990 ). The increased cardiac work due to higher heart rates would likely deplete cardiac glycogen, contributing to earlier decompensation ( Bennet, 2017 ). Blood pressure was slower to rise in the FGR group compared to controls, suggesting altered peripheral vascular resistance.
Consistent with this, chronically hypoxic fetal sheep raised at altitude and exposed to an acute episode of isocapnic hypoxia had a reduced capacity to induce peripheral vasoconstriction in order to maintain centralization of blood flow ( Herrera et al., 2016 ). In this study, chronically hypoxic fetuses did not exhibit classical changes in heart rate, blood pressure or peripheral blood flow during superimposed hypoxia. This might be due to impaired chemoreflex defenses or more simply to an altered homeostatic set point, given that the relative change from basal oxygenation was smaller in the FGR group. Similar high-altitude models of FGR have found that early-onset chronic fetal hypoxia alters vascular structure, vasoreactivity and cardiac function ( Pulgar et al., 2009 ; Ducsay et al., 2018 ). The impact of altered vascular function was also noted in a late-onset FGR model induced by sheep living in an isocapnic hypoxia chamber from ∼121 days of gestation for 10 days ( Allison et al., 2020 ). The chronically hypoxic fetuses exposed to acute hypotension demonstrated an impaired ability to restore blood pressure homeostasis, due to an absent baroreflex increase in fetal heart rate and altered peripheral vascular sensitivity to adrenergic stimulation. An earlier fetal sheep study of prolonged partial cord compression between 125 and 128 days of gestation, followed by episodes of acute hypoxemia, suggests the fetal response relies less on peripheral vasoconstriction and depends more on umbilical vasodilation in order to maintain perfusion to essential beds ( Gardner and Giussani, 2003 ).
These studies represent different paradigms of FGR, with different degrees and duration of hypoxia. It should further be noted that while an acute moderate isocapnic challenge allows assessment of the fetal chemoreflex, it does not represent a real-life hypoxia challenge. Overall, combined with the studies of spontaneous hypoxia discussed above, these studies suggest that FGR per se does not impair the initial chemoreflex defense responses to hypoxia, but that these critical responses may not be sustained in many fetuses, leading to earlier and potentially terminal decompensation. Clinically, the difficulty detecting FGR, particularly later-onset FGR, impedes studies of the impact of birth asphyxia in FGR. Moreover, 97% of deliveries in the TRUFFLE study of early-onset FGR were by Cesarean section ( Lees et al., 2013 ), and so precluded labor-induced asphyxia. There are few clinical data regarding the effects of comorbid FGR on birth asphyxia.
Thus, timely detection of FGR and at-risk fetuses is vital to reduce adverse outcomes ( Baschat, 2018 ; McCowan et al., 2018 ; Ego et al., 2020 ). Detection of FGR alone can reduce the risk of stillbirth by nearly 40% as observed in a study of 92,182 births at ≥22 weeks of gestation ( Ego et al., 2020 ). Effective monitoring of FGR progression is critical to balance the risk of worsening fetal oxygen deprivation and stillbirth against the risks of neonatal complications after preterm birth if the infant is delivered ( Baschat, 2018 ; Delnord and Zeitlin, 2019 ). To improve outcomes, we need reliable biomarkers to identify FGR and to monitor evolving risks of deteriorating oxygenation or impact on neural development. Figure 1 summarizes current detection and monitoring techniques. Below, we briefly discuss current monitoring techniques, and advances in imaging and fetal heart rate monitoring.
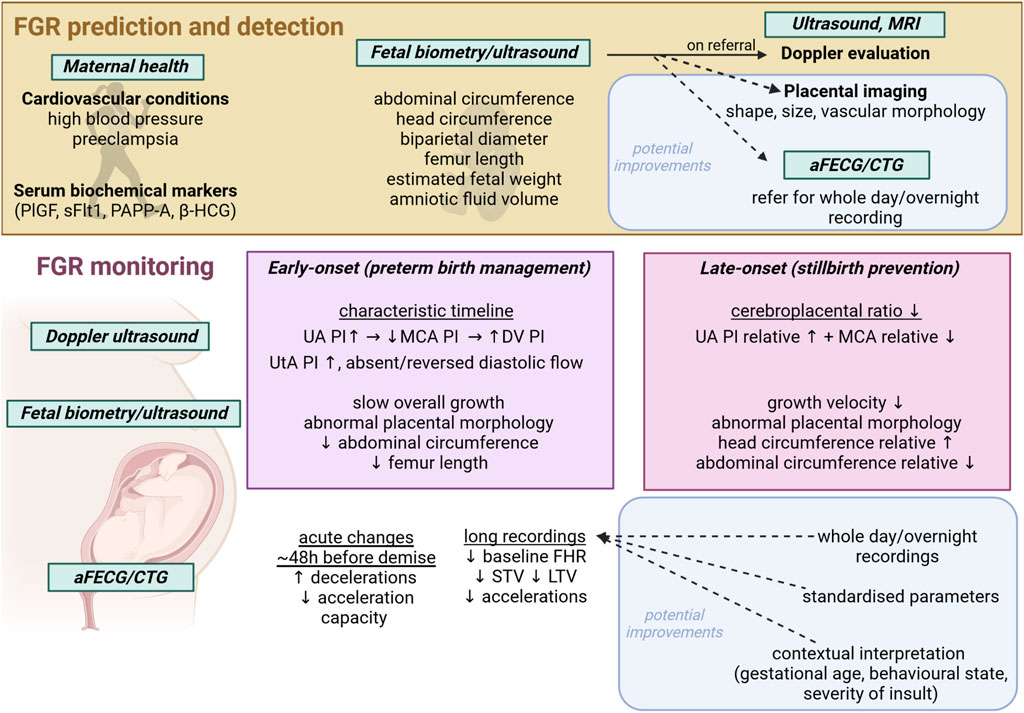
FIGURE 1 . Schematic showing current FGR detection and monitoring techniques and modalities, with potential avenues for improvement. aFECG, abdominal fetal electrocardiogram; CTG, cardiotocogram; MRI, magnetic resonance imaging; UA, umbilical artery; MCA, middle cerebral artery; DV, ductus venosus; PI, pulsatility index; STV, short term variability; LTV, long term variability; PlGF, placental growth factor; sFlt1, soluble fms-like tyrosine kinase-1; PAPP-A, pregnancy associated plasma protein A; β-HCG, beta human chorionic gonadotropin; SPINT, serine protease inhibitor.
Doppler ultrasound, fetal biometry and biochemistry measures
Doppler ultrasound is routinely used to assess the hemodynamic aspects of placental dysfunction, as reviewed in detail ( Vollgraff Heidweiller-Schreurs et al., 2018 ; Smith et al., 2021 ; Dall'Asta et al., 2022 ). Early-onset FGR is associated with abnormal umbilical and uterine vessel Doppler findings ( Mifsud and Sebire, 2014 ; Baschat, 2018 ; Figueras et al., 2018 ; Lees et al., 2022 ) ( see Table 1 ). In this setting, the umbilical artery (UA) waveform shows an increased pulsatility index (PI), and absent or reversed end diastolic velocities that can worsen as pregnancy progresses with increasing placental flow resistance ( Cohen et al., 2016 ; Baschat, 2018 ; Caradeux et al., 2018 ; Morales-Roselló et al., 2018 ). The rate of increase in placental blood flow resistance is the primary determinant of early FGR progression and risk of fetal deterioration. A recent meta-analysis reported that the odds ratio of fetal death associated with early-onset FGR were 3.59 and 7.27 with absent and reversed UA end diastolic velocities, respectively ( Caradeux et al., 2018 ). Furthermore, the extent of absent end diastolic velocity in the cardiac cycle was shown to be predictive of the risk of fetal death ( Kinoshita et al., 2021 ). Similarly, absent and reversed ductus venosus flow are associated with worsening placental dysfunction and impaired fetal cardiac function. In early-onset FGR with absent or reversed end diastolic UA flow, abnormal ductus venosus Doppler measures were associated with further increased risk of stillbirth ( Caradeux et al., 2018 ).
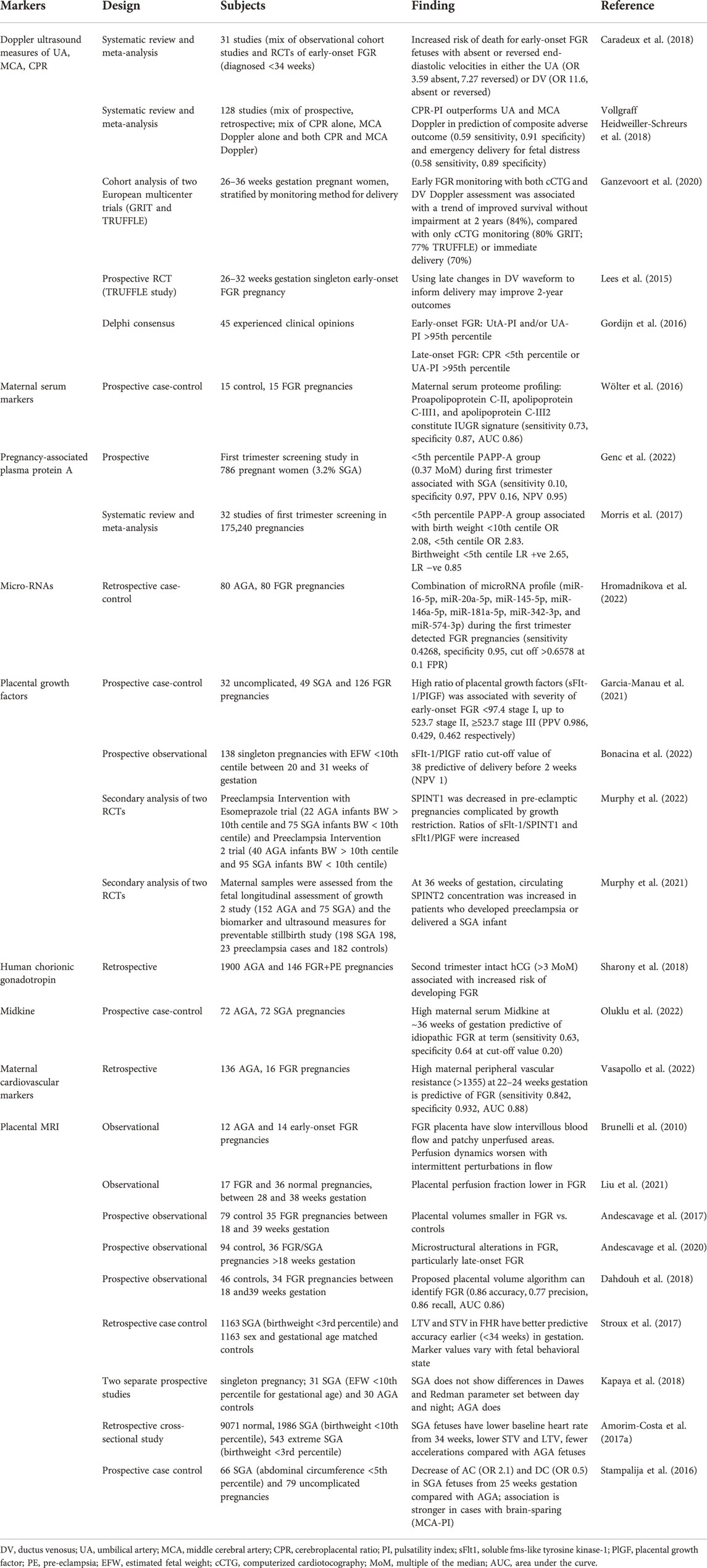
TABLE 1 . Summary of select studies of potential biochemical and physiological markers of FGR.
The majority of cases of FGR are late-onset and more difficult to detect as they present with more subtle uterine or umbilical Doppler changes, or even with apparently normal flows, despite histological evidence of compromise in the placenta ( Mifsud and Sebire, 2014 ; Baschat, 2018 ; Figueras et al., 2018 ; Dall'Asta et al., 2022 ; Lees et al., 2022 ). Redistribution of fetal combined ventricular output, with increased perfusion to the brain and associated brain-sparing, is a key indicator of the presence of fetal hypoxemia and adaptation. Thus, Doppler measurements of the middle cerebral artery (MCA) provide some utility in identifying at risk fetuses. Abnormal MCA Doppler measures in in later-onset FGR may, for example, be associated with increased risk of stillbirth within the next 4–7 days ( Baschat, 2018 ). It is recognized that the difference between the pulsatility index of the UA and MCA (the cerebroplacental ratio, CPR) is more sensitive than either alone ( Kalafat and Khalil, 2018 ; Vollgraff Heidweiller-Schreurs et al., 2018 ; Ciobanu et al., 2019 ). An abnormal CPR is associated with increased risk of stillbirth, and neonatal morbidity including adverse neurodevelopmental outcomes in survivors ( Crimmins et al., 2014 ; Khalil and Thilaganathan, 2017 ; Kalafat et al., 2019 ; Monteith et al., 2019 ). It may also discriminate between SGA and FGR fetuses in cases of poor maternal vascular perfusion placental pathologies, but is reported to have limited utility for excluding FGR in other types of placental pathologies (i.e. fetal vascular malperfusion, or villitis of unknown etiology) ( Ashwal et al., 2022 ).
However, like MCA measures, CPR alone remains a relatively poor predictor of perinatal outcomes in isolation, and combined measures are more predictive ( Dall'Asta et al., 2019 ; D'Antonio et al., 2020 ; Schiffer et al., 2021 ; Vollgraff Heidweiller-Schreurs et al., 2021 ). There is a need for gestational age-based reference ranges for the pulsatility index from all vascular beds ( Ciobanu et al., 2019 ). Fetal biometric information, such as ultrasound measures of abdominal circumference and femur length, are used to estimate fetal weight and provide additional information ( Kiserud et al., 2017 ; Hiersch and Melamed, 2018 ). Some evidence suggests that universal third trimester ultrasound tripled SGA infant detection, and combining this approach with Doppler velocimetry measures identified SGA infants at high risk of morbidity ( Sovio and Smith, 2018 ). Worryingly, other studies have shown that diagnosis of FGR by third trimester ultrasound did not materially improve perinatal outcomes ( Henrichs et al., 2019 ; Bonnevier et al., 2022 ). Some of this discrepancy may be due to different gestational ages at screening ( Roma et al., 2015 ), which highlights the importance of timing in the interpretation of biomarkers. Further, in many clinical settings misdiagnoses can continue due to inconsistencies in reference charts and reporting ( Paoletti et al., 2021 ).
Doppler measures and biometry in tandem with plasma biomarker factors is proposed to increase sensitivity to distinguish FGR from SGA and AGA and those fetuses at greater risk of adverse events, thereby guiding clinical decision making around extra monitoring and early delivery ( Wölter et al., 2016 ; Sovio et al., 2020 ). A combination approach incorporating maternal biochemical markers with fetoplacental ultrasound measures produces moderate improvements in FGR detection over either technique alone ( Miranda et al., 2017 ), and may be able to distinguish between early- and later-onset phenotypes ( Crovetto et al., 2016 ). However, the evidence is inconsistent ( McCowan et al., 2017 ) and presently, use of maternal serum markers is not recommended for routine screening due to a lack of evidence ( Heazell et al., 2015 ).
These tools have been used to directly investigate the concept that clinical FGR may impair fetal responses. For example, during an oxytocin challenge, fetuses with suspected FGR >36 weeks gestation, without evidence of brain-sparing ( Fu and Olofsson, 2006 ), were able to increase cerebral perfusion during oxytocin, whereas fetuses with established brain sparing showed little change, suggesting impaired ability to respond to an acute challenge.
Plasma and maternal cardiovascular and serum biomarkers
Placental factors are released into maternal circulation from the early stages of placentation, and some factors change with altered placental growth, function and impaired oxygenation and/or damage to the placenta ( Araujo Junior et al., 2021 ). A recent metabolomic study demonstrated high predictive accuracy for FGR with a combination of 3-hydroxybutyric acid, glycine and phosphatidylcholine with acyl-alkyl residue C42 ( Bahado-Singh et al., 2022 ). Lipoproteins in maternal serum may have discriminatory value for distinguishing FGR from AGA pregnancy ( Wölter et al., 2016 ), aligning with evidence for a maternal component in altered lipid metabolism in FGR pregnancy ( Paules et al., 2020 ). Several potential predictors of placental dysfunction have been identified during first and second trimesters, including pregnancy associated plasma protein A, β-human chorionic gonadotropin, tyrosine kinase-1 and 2, hypoxia-inducible factors 1–3, vascular endothelial growth factors, placental growth factor, angiopoietin-1 and -2, soluble fms-like tyrosine kinase-1, placental growth factor and serine protease inhibitor ( Audette and Kingdom, 2018 ; Sun et al., 2020 ; Murphy et al., 2021 ; Smith, 2021 ; Bahado-Singh et al., 2022 ).
Table 1 provides a summary of biochemical biomarkers of placental dysfunction and FGR. Used alone, however, such markers have poor positive predictive value for FGR and poor outcome ( Conde-Agudelo et al., 2013 ). Additionally, many studies examine a cross-section of fetuses at a single-time-point, which may miss later-onset FGR, and makes it difficult to assess the modulating effect of gestational age ( Pecks et al., 2016 ). Large scale, longitudinal trials are required to determine validity and specificity of biomarkers for FGR and risk of adverse events.
Altered maternal hemodynamics play a key role in the pathophysiology of FGR. In particular, maternal hypertension and altered peripheral vascular resistance are the hallmarks of pre-eclampsia, which is strongly associated with FGR ( Kametas et al., 2022 ). There is now interest in whether general measures of maternal hemodynamics can be used to discriminate between FGR and SGA and to determine fetal risks. Perry and others have shown that lower maternal heart rate and cardiac output, higher blood pressure and greater peripheral and uterine artery resistance may distinguish between SGA and FGR fetuses ( Perry et al., 2020 ). Increased peripheral vascular resistance in mothers with chronic hypertension is highly predictive of birthweight and FGR by mid-pregnancy ( Vasapollo et al., 2022 ), and lower cardiac output and increased systemic resistance was observed with FGR. These parameters were also a strong indicator of subsequent neonatal hospitalization ( Di Pasquo et al., 2021 ). Reduced maternal perfusion can lead to lesions related to hypoxia. However, the presence of placental lesions per se is not predictive of FGR ( Ferrazzi et al., 1999 ; Figueras et al., 2009 ). These studies have not tracked longitudinal changes in function, and so FGR characterized by reduced growth velocity rather than Dephi consensus criteria ( Kennedy et al., 2020 ; Molina et al., 2020 ) could have been miscategorized as a control pregnancy. Secondary applications of algorithms for predicting early-onset pre-eclampsia have been tested for their capacity to predict SGA at birth ( Mendoza et al., 2022 ). Overall, they have an overall poor predictive value ( Crovetto et al., 2017 ), preventing current use in screening a “low-risk” population ( Blitz et al., 2016 ).
Given that increased vascular resistance and reduced fetoplacental blood flow are key factors in the mediating FGR, there is a need to better evaluate maternal and placental perfusion at the macro and micro level, using advanced approaches to ultrasound measurements and utilizing MRI, which allows more in-depth assessment of vascular structure, oxygenation and blood flow to provide insight into functionality ( Aughwane et al., 2021 ; Schiffer et al., 2021 ; Clark et al., 2022a ) ( see Figure 2 for example). For instance, Clark and others have demonstrated that investigators need to evaluate the utero-placental vasculature as a whole, rather than just the spiral arteries, in order to better interpret uterine waveforms ( Clark et al., 2018 ). Computational, biomechanical analysis may improve reliability of these measures ( Clark et al., 2022b ). Substantial work is still needed to validate both advanced ultrasound and MRI techniques experimentally and clinically ( Andescavage and Limperopoulos, 2021 ; Saini et al., 2021 ; Clark et al., 2022a ). Current data have shown that multi-compartment MRI can be used to evaluate local blood volumes within the placenta and fetoplacental blood oxygenation, and may be able to detect differences between FGR and normal pregnancies ( Aughwane et al., 2021 ; Jacquier and Salomon, 2021 ). MRI can detect changes in the placentas of severe FGR fetuses with abnormal Doppler velocimetry ( Ingram et al., 2017 ; Aughwane et al., 2021 ) and T2* values (which reflect, in part, oxyhemoglobin) are reportedly lower only in FGR cases with abnormal Doppler and where there is histological evidence of maternal hypoperfusion ( Sinding et al., 2016 ). Placental pathologies have been shown in women with chronic hypertension using combined placental MR examination (e.g. T2 weighted imaging, T2*, T1 mapping and diffusion imaging ( Ho et al., 2021 )), and defined assessment templates using combined MR measurements allow for discrimination of normal and abnormal placental development ( Ho et al., 2022 ). Diffusion weighted imaging has shown reduced placental perfusion in FGR pregnancies; Intravoxel Incoherent Motion (IVIM) models applied to MRI allow quantification of microstructure and blood flow without using contrast agents, and studies have shown utility in determining micro-vascularization changes in FGR pregnancies ( Liu et al., 2021 ; Antonelli et al., 2022 ) without any sign of Doppler ultrasound impairment ( Antonelli et al., 2022 ). Gestational age-associated changes in placental IVIM parameters likely reveal trajectories of microvascular perfusion fraction and diffusion characteristics in the developing placenta.
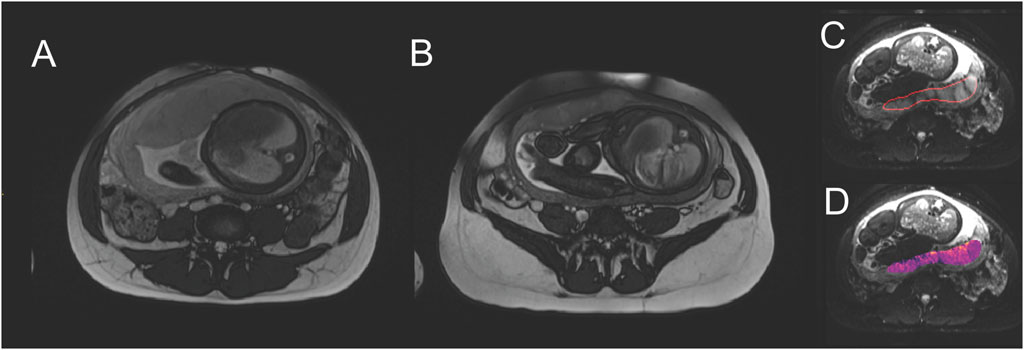
FIGURE 2 . Example of placental MRI modalities. Comparing central slices through the placenta, (A) is a normal pregnancy (fetal abdominal diameter 96.5 mm) at 37 weeks gestation, with placental thickness of 41.2 mm. (B) is FGR (fetal abdominal diameter 79.2 mm, birthweight 1.4 centile) at 37 weeks gestation, with placental thickness of 21.8 mm. Placentae in FGR pregnancies are typically described as smaller and appearing darker and more heterogenous in MRI. (C) shows identification of a placental region of interest. (D) shows a parameter map of apparent diffusion coefficient, a parameter that relates to blood movement, and is typically extracted from MRI studies.
While MRI can provide valuable insights into fetal and placental disease progression in FGR ( Kingdom et al., 2018 ), it is not particularly suitable as a screening technique as it demands intensive resources. Thus, we continue to seek other more generic monitoring modalities, that can be used serially and without expensive or intensive resources. In this regard, the final part of our review looks at antenatal fetal cardiotocography (CTG) used to derive fetal heart rate and other indices.
Antenatal fetal heart rate and heart rate variability markers
In current practice, early-onset FGR is assessed with Doppler ultrasound and FHR variability (FHRV) measurements. Antenatal cardiotocography (CTG) involves monitoring fetal heart rate and uterine contractions by ultrasound. Changes in FHR reflect changes in fetal oxygen level, autonomic nervous system function, cardiovascular maturation and neurodevelopment, as behavioral state (the emergence of which is a key neurodevelopmental milestone) ( Hoyer et al., 2017 ). Overt FHR abnormalities are largely only observed during a very short terminal phase. This may be preceded by a gradual reduction in FHRV, but in practice, marked suppression of short-term variability (STV) is only reliably seen in the terminal stage of FGR in the final 1–2 days before fetal demise or appropriate delivery ( Baschat et al., 2022 ). At this terminal stage, suppressed STV may also be associated with FHR decelerations. This combination was observed in 44% of cases just before delivery in the Trial of Umbilical and Fetal Flow in Europe (TRUFFLE) study ( Lees et al., 2015 ). This is consistent with the hypothesis that this pattern represents the terminal stage of failing uteroplacental function. Thus, current methods are both highly stressful to the family, as they require daily monitoring, and yet are still associated with only 77%–85% survival without neurodevelopmental impairment ( Baschat et al., 2022 ).
Improving FHR monitoring for FGR detection and monitoring
The time period and timing of recording is highly important in the interpretation of FHRV. This is due to varying fetal behavioral states. Before 32 weeks gestation, fetuses show cycles of quiescence and activity ( Keen et al., 2011 ). In preterm life, fetal activity, such as breathing-like movements, body movements, sucking, yawning, swallowing is nearly continuous. From around 32 weeks gestation, distinct behavioral sleep states that were first identified in newborns and then detected by FHR in the fetus, begin to emerge ( Keen et al., 2011 ). With maturation, the fetus spends about two-thirds of the time in active sleep (2F, characterized by a steady FHR, eye movements, and frequent body movements), a quarter in quiet sleep (1F, characterized by slow, steady FHR and few body movements) and the remainder in 4F (so-called “active awake,” characterized by fast, irregular FHR and continual eye and body movements) ( Brändle et al., 2015 ). The reader should note that despite the terminology there is no evidence that the fetus is actually awake in any of these states ( Mellor et al., 2005 ).
Preterm fetal sheep show a discontinuous EEG, containing a mixture of frequencies and amplitudes. As the fetus develops towards term, the EEG becomes continuous, with distinct low-frequency-high-amplitude (REM) and high-frequency-low amplitude (NREM) sleep states ( Dawes et al., 1972 ; Richardson et al., 1994 ). REM states contain breathing movements and eye movements; NREM additionally has body movements ( Szeto and Hinman, 1985 ). These EEG and behavioral patterns and their development are similarly seen in monitored preterm-born and term-born humans ( Sandoval Karamian and Wusthoff, 2021 ) (for review, see ( Bennet et al., 2018 )).
Thus, FHR and derived indices can vary considerably depending on fetal behavioral state ( Signorini et al., 2003 ). Therefore, differences in fetal sleep-state during recording can confound comparisons of FHRV measurements ( Sriram et al., 2013 ). Prolonged fetal FHR recording would be required to capture both active and quiet sleep, which would require recording for 2 h or longer ( Ryo et al., 2018 ; Sumiyoshi et al., 2020 ), and a calculated average may be used for comparison ( Signorini et al., 2020 ). Additionally, sleep state development is altered with fetal hypoxemia ( Boddy et al., 1974 ; Lee et al., 2009 ). Consistent with the impaired neurodevelopment of the chronically hypoxic fetus, in daily FHR recordings which began between 24 and 28 weeks gestation, SGA fetuses (EFW <10th percentile) were found to have a delay in FHR rhythm formation, indicative of delayed fetal behavioral state differentiation/sleep-state cycling formation, of around 1–2 weeks ( Sumiyoshi et al., 2020 ). Further, a reduction in fetal movements is associated with fetal hypoxemia and FGR ( Heazell and Frøen, 2008 ). Placental dysfunction and insufficient fetal oxygenation have been linked with reduced fetal movements ( Ter Kuile et al., 2021 ), which is thought to be an oxygen conservation strategy ( Warrander and Heazell, 2011 ). A combined assessment of FHRV with sleep-state pattern (derived as an index of fetal activity from the presence of accelerations and long term variability measures) can improve detection of FGR ( Stroux et al., 2017 ).
Similarly, there are known circadian rhythms of FHR and variability; therefore, the timing of measurement may be important ( Kapaya et al., 2016 ). Fetuses receive circadian cues from their mothers and have circadian rhythms in both physiological and pathophysiological conditions. Circadian rhythms in FHR have been observed as early as 20 weeks of gestation in human pregnancies ( de Vries et al., 1987 ). FHR is known to increase in the late afternoon, peaking in the early-mid evening; in part, these measures reflect fetal behavior and the circadian timing of fetal movements ( Bradford et al., 2019 ). Despite its limited predictive value, intermittent auscultation is standard practice to monitor FHR ( Henderson et al., 2000 ; Haws et al., 2009 ; Murray, 2017 ). Clinical FHR monitoring is often performed indirectly using Doppler-based techniques; this necessarily limits monitoring time length and indeed, timing of monitoring, with sessions lasting 20–40 min ( Murray, 2017 ). More frequent monitoring is performed only where clinical concern is identified, and sessions are relatively short, far less than a whole circadian period. The growth and circadian rhythmicity of normally developing fetuses is sufficient to produce conflicting results when measurements are taken day-on-day, and within a day measurements vary substantially, reflecting changing fetal behaviors ( Lunshof et al., 1998 ; Babazadeh et al., 2005 ; Avitan et al., 2018 ).
Short duration recordings during prenatal visits do not capture sufficient data for these analyses ( Ryo et al., 2018 ). Therefore, using an ECG for overnight fetal monitoring has gained interest for monitoring of the fetus. A study of FHR recordings for 20 h from singleton pregnancies (30 AGA and 31 SGA) reported that SGA fetuses had a blunted diurnal rhythm in STV, LTV, accelerations and time spent in high frequency activity, although the absolute values were within normal range ( Kapaya et al., 2018 ). These findings suggest that the time of day can affect the interpretation of FHR and FHRV measures, and further studies are required to assess the diagnostic potential of diurnal change in FHR measures to identify FGR.
In addition to changes with fetal sleep-state and circadian pattern, there are maturational changes in FHR and FHRV over the course of gestation. Surprisingly, the majority of studies of CTG parameters in FGR fetuses did not control for gestational age, despite including fetuses from a range of gestation ages. A longitudinal study of 176 pregnancies (31 SGA) using sequential CTG recordings from 24 to 39 weeks of gestation reported that with advancing gestation age both SGA and AGA fetuses had a reduction in baseline FHR and increase in short-term and long-term HRV (Amorim-Costa et al.). However, SGA fetuses had a much steeper descent in baseline FHR and lower LTV than AGA ( Amorim-Costa et al., 2017b ). Similarly, a cross-sectional CTG study in a large cohort (9701 AGA and 1986 SGA (birth weight <10th percentile for gestational age)) reported that SGA fetuses had a lower baseline heart rate from 34 weeks of gestation, and lower average STV and LTV and fewer accelerations compared with AGA fetuses ( Amorim-Costa et al., 2017a ). These longitudinal differences in FGR may reflect relatively greater parasympathetic influence ( Vinkesteijn et al., 2004 ; Shaw et al., 2018 ). Future studies assessing sequential changes in FHR and FHRV parameters in conjunction with fetal Doppler measures over gestation, and correlating these with fetal outcomes, will help to evaluate if these markers can be used for detection and management of FGR fetuses.
FGR fetuses are generally believed to be able to compensate for stable chronic hypoxemia-, and so they may have reduced physiological reserves to withstand additional insults as discussed earlier. Maternal supine sleep position is associated with fetal growth restriction and is an independent risk factor for late gestation stillbirth ( Gordon et al., 2015 ). Notably, maternal obstructive sleep apnea has been associated with reduced fetal growth velocity ( Fung et al., 2013 ; Kneitel et al., 2018 ). Sleep position can also alter maternal hemodynamics. A study using MRI in women in the third trimester of pregnancy found that the maternal supine position is associated with a 32% reduction in aorta flow and 85% reduction in flow through the inferior vena cava, suggesting increased reliance on collateral venous circulation to maintain cardiac output ( Humphries et al., 2018 ). Moreover, maternal sleep position was associated with changes in fetal behavioral state and FHR variability ( Stone et al., 2017 ). A study of CTG with maternal polysomnography in late pregnancy reported that nocturnal FHR decelerations and prolonged reduction in FHRV were more likely in pregnancies complicated by hypertension and/or FGR, and these changes were associated with maternal sleep position ( Wilson et al., 2022 ). This suggests that an adverse event (e.g. a single apnea episode, or single position change) may initiate/provoke a hypoxic challenge, the response to which may be altered depending on extant fetal capacity and autonomic maturation ( Robertson et al., 2019 ). Overnight observations might provide insight into the extent of fetal (de)compensation in FGR.
In FGR with brain-sparing, accelerative and decelerative capacity (AC and DC) are reduced from 25 weeks gestation to term, compared with normal fetuses ( Stampalija et al., 2016 ; Frasch et al., 2018 ). In prenatal ambulatory CTG recordings (mean length 42 min) AC was able to distinguish between normal and FGR pregnancies, as was STV ( Huhn et al., 2011 ). An observational study of overnight FHR recordings in normal and SGA pregnancies, STV, found that average AC and average DC were significantly lower in SGA pregnancy, though these parameters were unable to distinguish between the heterogeneous etiologies in the SGA group ( Graatsma et al., 2012 ). While longer recordings are desirable for capture of relevant behavioral states in later gestation, even short recordings may have some value earlier in gestation. A retrospective analysis of 3min segments of FHR recordings taken from 28 weeks gestation onwards found that time-domain measures were able to discriminate between normal and FGR fetuses, prior to 36 weeks gestation ( Esposito et al., 2019 ). It may be that such measures would be of most use before 34 weeks gestation ( Stampalija et al., 2015 ; Tagliaferri et al., 2015 ). However, the recordings analyzed here are usually taken in the context of pre-selected groups, limiting the application of the findings with a view to create a screening tool. It is likely that a combinatorial approach to screening may offer the best predictive capacity to identify at-risk fetuses; for example, a study found that the combination of Doppler velocimetry and scheduled computerized CTG analysis was reasonably effective in preventing fetal demise in early-onset FGR ( Wolf et al., 2020 ). Current efforts to generate a set of FHR-derived indices for screening purposes reiterate the need to incorporate factors such as gestational age and insult severity ( Hoyer et al., 2019 ). Machine-learning approaches in addition to hypothesis-driven approaches are helping to identify and consolidate measures which might best discriminate the at-risk fetus ( Signorini et al., 2020 ; Khatibi et al., 2021 ; Pini et al., 2021 ).
Current screening techniques do not provide good detection rates for fetal growth restriction, and later-onset growth restriction in particular. Once detected, present monitoring strategies are not sufficient to provide timely and reliable detection of fetal compromise in FGR. There is urgent need to improve detection and monitoring of FGR to improve outcomes. Measures of fetal heart rate and variability are readily accessible and easily deployed in low-resource settings, but short-term recordings limit their utility. A thorough exploration of the efficacy of these measures requires research using longer, more frequent recordings. A better understanding of the utility of monitoring variation in FHR and derived indices with fetal behavioral state, circadian timing and maturation in FGR pregnancies is important. Advanced imaging techniques for assessing placental structure and function are also emerging as a promising tool for FGR detection. Preclinical studies with standardized animal models and computational modelling work will allow for deeper investigation of disease progression and biomarker identification. Once identified, suitable biomarker candidates could be incorporated in a combinatorial approach to a detection framework.
Author contributions
SD, VK, and LB conceptualized this review. SD, VK, AC, PR, AG, and LB undertook manuscript writing and figure preparation. All authors reviewed and edited this manuscript.
This work was supported by the Health Research Council of New Zealand, grant numbers 17/601 and 20/437, Cure Kids, Grant Number 3720229 and the Auckland Medical Research Foundation, Grant Number 1118010. VK was supported by a University of Auckland Doctoral Scholarship.
Acknowledgments
Figure 1 created with BioRender.com .
Conflict of interest
The authors declare that the research was conducted in the absence of any commercial or financial relationships that could be construed as a potential conflict of interest.
Publisher’s note
All claims expressed in this article are solely those of the authors and do not necessarily represent those of their affiliated organizations, or those of the publisher, the editors and the reviewers. Any product that may be evaluated in this article, or claim that may be made by its manufacturer, is not guaranteed or endorsed by the publisher.
Allison B. J., Brain K. L., Niu Y., Kane A. D., Herrera E. A., Thakor A. S., et al. (2020). Altered cardiovascular defense to hypotensive stress in the chronically hypoxic fetus. Hypertension 76 (4), 1195–1207. doi:10.1161/HYPERTENSIONAHA.120.15384
PubMed Abstract | CrossRef Full Text | Google Scholar
Amaya K. E., Matushewski B., Durosier L. D., Frasch M. G., Richardson B. S., Ross M. G. (2016). Accelerated acidosis in response to variable fetal heart rate decelerations in chronically hypoxic ovine fetuses. Am. J. Obstet. Gynecol. 214 (2), 270. doi:10.1016/j.ajog.2015.09.084
Amorim-Costa C., de Campos D. A., Bernardes J. (2017a). Cardiotocographic parameters in small-for-gestational-age fetuses: How do they vary from normal at different gestational ages? A study of 11687 fetuses from 25 to 40 weeks of pregnancy. J. Obstet. Gynaecol. Res. 43 (3), 476–485. doi:10.1111/jog.13235
Amorim-Costa C., Gaio A. R., Ayres-de-Campos D., Bernardes J. (2017b). Longitudinal changes of cardiotocographic parameters throughout pregnancy: A prospective cohort study comparing small-for-gestational-age and normal fetuses from 24 to 40 weeks. J. Perinat. Med. 45 (4), 493–501. doi:10.1515/jpm-2016-0065
Anderson N. H., Sadler L. C., McKinlay C. J. D., McCowan L. M. E. (2016). INTERGROWTH-21st vs customized birthweight standards for identification of perinatal mortality and morbidity. Am. J. Obstet. Gynecol. 214 (4), 509. doi:10.1016/j.ajog.2015.10.931
Andescavage N., duPlessis A., Metzler M., Bulas D., Vezina G., Jacobs M., et al. (2017). In vivo assessment of placental and brain volumes in growth-restricted fetuses with and without fetal Doppler changes using quantitative 3D MRI. J. Perinatol. 37 (12), 1278–1284. doi:10.1038/jp.2017.129
Andescavage N., Limperopoulos C. (2021). Emerging placental biomarkers of health and disease through advanced magnetic resonance imaging (MRI). Exp. Neurol. 347, 113868. doi:10.1016/j.expneurol.2021.113868
Andescavage N., You W., Jacobs M., Kapse K., Quistorff J., Bulas D., et al. (2020). Exploring in vivo placental microstructure in healthy and growth-restricted pregnancies through diffusion-weighted magnetic resonance imaging. Placenta 93, 113–118. doi:10.1016/j.placenta.2020.03.004
Antonelli A., Capuani S., Ercolani G., Dolciami M., Ciulla S., Celli V., et al. (2022). Human placental microperfusion and microstructural assessment by intra-voxel incoherent motion MRI for discriminating intrauterine growth restriction: A pilot study. J. Maternal-Fetal Neonatal Med. 2022, 1–8. doi:10.1080/14767058.2022.2050365
CrossRef Full Text | Google Scholar
Araujo Junior E., Zamarian A. C., Caetano A. C., Peixoto A. B., Nardozza L. M. (2021). Physiopathology of late-onset fetal growth restriction. Minerva Obstet. Gynecol. 73 (4), 392–408. doi:10.23736/S2724-606X.21.04771-7
Ashwal E., Ferreira F., Mei-Dan E., Aviram A., Sherman C., Zaltz A., et al. (2022). The accuracy of Fetoplacental Doppler in distinguishing between growth restricted and constitutionally small fetuses. Placenta 120, 40–48. doi:10.1016/j.placenta.2022.02.007
Audette M. C., Kingdom J. C. (2018). Screening for fetal growth restriction and placental insufficiency. Semin. Fetal Neonatal Med. 23 (2), 119–125. doi:10.1016/j.siny.2017.11.004
Aughwane R., Mufti N., Flouri D., Maksym K., Spencer R., Sokolska M., et al. (2021). Magnetic resonance imaging measurement of placental perfusion and oxygen saturation in early-onset fetal growth restriction. Bjog 128 (2), 337–345. doi:10.1111/1471-0528.16387
Avitan T., Sanders A., Brain U., Rurak D., Oberlander T. F., Lim K. (2018). Variations from morning to afternoon of middle cerebral and umbilical artery blood flow, and fetal heart rate variability, and fetal characteristics in the normally developing fetus. J. Clin. Ultrasound. 46 (4), 235–240. doi:10.1002/jcu.22569
Babazadeh R., Abdali K., Lotfalizadeh M., Tabatabaie H. R., Kaviani M. (2005). Diurnal nonstress test variations in the human fetus at risk. Int. J. Gynaecol. Obstet. 90 (3), 189–192. doi:10.1016/j.ijgo.2005.05.011
Bahado-Singh R. O., Turkoglu O., Yilmaz A., Kumar P., Zeb A., Konda S., et al. (2022). Metabolomic identification of placental alterations in fetal growth restriction. J. Matern. Fetal. Neonatal Med. 35 (3), 447–456. doi:10.1080/14767058.2020.1722632
Baschat A. A., Galan H. L., Lee W., DeVore G. R., Mari G., Hobbins J., et al. (2022). The role of the fetal biophysical profile in the management of fetal growth restriction. Am. J. Obstet. Gynecol. 226 (4), 475–486. doi:10.1016/j.ajog.2022.01.020
Baschat A. A. (2018). Planning management and delivery of the growth-restricted fetus. Best. Pract. Res. Clin. Obstet. Gynaecol. 49, 53–65. doi:10.1016/j.bpobgyn.2018.02.009
Benítez-Marín M. J., Marín-Clavijo J., Blanco-Elena J. A., Jiménez-López J., González-Mesa E. (2021). Brain sparing effect on neurodevelopment in children with intrauterine growth restriction: A systematic review. Children 8 (9), 745. doi:10.3390/children8090745
Bennet L., Booth L. C., Ahmed-Nasef N., Dean J. M., Davidson J., Quaedackers J. S., et al. (2007). Male disadvantage? Fetal sex and cardiovascular responses to asphyxia in preterm fetal sheep. Am. J. Physiol. Regul. Integr. Comp. Physiol. 293 (3), R1280–R1286. doi:10.1152/ajpregu.00342.2007
Bennet L. (2017). Sex, drugs and rock and roll: Tales from preterm fetal life. J. Physiol. 595 (6), 1865–1881. doi:10.1113/JP272999
Bennet L., Walker D. W., Horne R. S. C. (2018). Waking up too early - the consequences of preterm birth on sleep development. J. Physiol. 596 (23), 5687–5708. doi:10.1113/JP274950
Bennet L., Westgate J. A., Liu Y. C., Wassink G., Gunn A. J. (2005). Fetal acidosis and hypotension during repeated umbilical cord occlusions are associated with enhanced chemoreflex responses in near-term fetal sheep. J. Appl. Physiol. 99 (4), 1477–1482. doi:10.1152/japplphysiol.00431.2005
Beune I. M., Bloomfield F. H., Ganzevoort W., Embleton N. D., Rozance P. J., van Wassenaer-Leemhuis A. G., et al. (2018). Consensus based definition of growth restriction in the newborn. J. Pediatr. 196, 71. doi:10.1016/j.jpeds.2017.12.059
Biks G. A., Blencowe H., Hardy V. P., Geremew B. M., Angaw D. A., Wagnew A., et al. (2021). Birthweight data completeness and quality in population-based surveys: EN-INDEPTH study. Popul. Health Metr. 19 (1), 17. doi:10.1186/s12963-020-00229-w
Binder J., Monaghan C., Thilaganathan B., Morales-Roselló J., Khalil A. (2018). Reduced fetal movements and cerebroplacental ratio: Evidence for worsening fetal hypoxemia. Ultrasound Obstet. Gynecol. 51 (3), 375–380. doi:10.1002/uog.18830
Blencowe H., Krasevec J., de Onis M., Black R. E., An X., Stevens G. A., et al. (2019). National, regional, and worldwide estimates of low birthweight in 2015, with trends from 2000: A systematic analysis. Lancet. Glob. Health 7 (7), e849–e860. doi:10.1016/S2214-109X(18)30565-5
Blitz M. J., Rochelson B., Vohra N. (2016). Maternal serum analytes as predictors of fetal growth restriction with different degrees of placental vascular dysfunction. Clin. Lab. Med. 36 (2), 353–367. doi:10.1016/j.cll.2016.01.006
Block B. S., Schlafer D. H., Wentworth R. A., Kreitzer L. A., Nathanielsz P. W. (1989). Intrauterine growth retardation and the circulatory responses to acute hypoxemia in fetal sheep. Am. J. Obstet. Gynecol. 161 (6), 1576–1579. doi:10.1016/0002-9378(89)90929-0
Block B. S., Schlafer D. H., Wentworth R. A., Kreitzer L. A., Nathanielsz P. W. (1990). Regional blood flow distribution in fetal sheep with intrauterine growth retardation produced by decreased umbilical placental perfusion. J. Dev. Physiol. 13 (2), 81–85.
PubMed Abstract | Google Scholar
Boddy K., Dawes G. S., Fisher R., Pinter S., Robinson J. S. (1974). Foetal respiratory movements, electrocortical and cardiovascular responses to hypoxaemia and hypercapnia in sheep. J. Physiol. 243 (3), 599–618. doi:10.1113/jphysiol.1974.sp010768
Bonacina E., Mendoza M., Farràs A., Garcia-Manau P., Serrano B., Hurtado I., et al. (2022). Angiogenic factors for planning fetal surveillance in fetal growth restriction and small-for-gestational-age fetuses: A prospective observational study. Bjog 2022. doi:10.1111/1471-0528.17151
Bonnevier A., Maršál K., Källén K. (2022). Detection and clinical outcome of small-for-gestational-age fetuses in the third trimester—a comparison between routine ultrasound examination and examination on indication. Acta Obstet. Gynecol. Scand. 101 (1), 102–110. doi:10.1111/aogs.14278
Botting K. J., Loke X. Y., Zhang S., Andersen J. B., Nyengaard J. R., Morrison J. L. (2018). IUGR decreases cardiomyocyte endowment and alters cardiac metabolism in a sex- and cause-of-IUGR-specific manner. Am. J. Physiol. Regul. Integr. Comp. Physiol. 315 (1), R48–r67. doi:10.1152/ajpregu.00180.2017
Bradford B. F., Cronin R. S., McKinlay C. J. D., Thompson J. M. D., Mitchell E. A., Stone P. R., et al. (2019). A diurnal fetal movement pattern: Findings from a cross-sectional study of maternally perceived fetal movements in the third trimester of pregnancy. PloS One 14 (6), e0217583. doi:10.1371/journal.pone.0217583
Brändle J., Preissl H., Draganova R., Ortiz E., Kagan K. O., Abele H., et al. (2015). Heart rate variability parameters and fetal movement complement fetal behavioral states detection via magnetography to monitor neurovegetative development. Front. Hum. Neurosci. 9, 147. doi:10.3389/fnhum.2015.00147
Brunelli R., Masselli G., Parasassi T., De Spirito M., Papi M., Perrone G., et al. (2010). Intervillous circulation in intra-uterine growth restriction. Correlation to fetal well being. Placenta 31 (12), 1051–1056. doi:10.1016/j.placenta.2010.09.004
Burton G. J., Jauniaux E. (2018). Pathophysiology of placental-derived fetal growth restriction. Am. J. Obstet. Gynecol. 218 (2S), S745–S761. doi:10.1016/j.ajog.2017.11.577
Caradeux J., Martinez-Portilla R. J., Basuki T. R., Kiserud T., Figueras F. (2018). Risk of fetal death in growth-restricted fetuses with umbilical and/or ductus venosus absent or reversed end-diastolic velocities before 34 weeks of gestation: A systematic review and meta-analysis. Am. J. Obstet. Gynecol. 218 (2), S774S774–S782.e21. doi:10.1016/j.ajog.2017.11.566
Ciobanu A., Wright A., Syngelaki A., Wright D., Akolekar R., Nicolaides K. H. (2019). Fetal Medicine Foundation reference ranges for umbilical artery and middle cerebral artery pulsatility index and cerebroplacental ratio. Ultrasound Obstet. Gynecol. 53 (4), 465–472. doi:10.1002/uog.20157
Clark A., Flouri D., Mufti N., James J., Clements E., Aughwane R., et al. (2022a20211). Developments in functional imaging of the placenta. Br. J. Radiol. 2022. doi:10.1259/bjr.20211010
Clark A. R., James J. L., Stevenson G. N., Collins S. L. (2018). Understanding abnormal uterine artery Doppler waveforms: A novel computational model to explore potential causes within the utero-placental vasculature. Placenta 66, 74–81. doi:10.1016/j.placenta.2018.05.001
Clark A. R., Yoshida K., Oyen M. L. (2022b). Computational modeling in pregnancy biomechanics research. J. Mech. Behav. Biomed. Mat. 128, 105099. doi:10.1016/j.jmbbm.2022.105099
Cohen E., Wong F. Y., Horne R. S., Yiallourou S. R. (2016). Intrauterine growth restriction: Impact on cardiovascular development and function throughout infancy. Pediatr. Res. 79 (6), 821–830. doi:10.1038/pr.2016.24
Conde-Agudelo A., Papageorghiou A., Kennedy S., Villar J. (2013). Novel biomarkers for predicting intrauterine growth restriction: A systematic review and meta-analysis. BJOG Int. J. Obstetrics Gynaecol. 120 (6), 681–694. doi:10.1111/1471-0528.12172
Crimmins S., Desai A., Block-Abraham D., Berg C., Gembruch U., Baschat A. A. (2014). A comparison of Doppler and biophysical findings between liveborn and stillborn growth-restricted fetuses. Am. J. Obstet. Gynecol. 211 (6), 669. doi:10.1016/j.ajog.2014.06.022
Crovetto F., Triunfo S., Crispi F., Rodriguez-Sureda V., Dominguez C., Figueras F., et al. (2017). Differential performance of first-trimester screening in predicting small-for-gestational-age neonate or fetal growth restriction. Ultrasound Obstet. Gynecol. 49 (3), 349–356. doi:10.1002/uog.15919
Crovetto F., Triunfo S., Crispi F., Rodriguez-Sureda V., Roma E., Dominguez C., et al. (2016). First-trimester screening with specific algorithms for early- and late-onset fetal growth restriction. Ultrasound Obstet. Gynecol. 48 (3), 340–348. doi:10.1002/uog.15879
D'Antonio F., Rizzo G., Gustapane S., Buca D., Flacco M. E., Martellucci C., et al. (2020). Diagnostic accuracy of Doppler ultrasound in predicting perinatal outcome in pregnancies at term: A prospective longitudinal study. Acta Obstet. Gynecol. Scand. 99 (1), 42–47. doi:10.1111/aogs.13705
Dahdouh S., Andescavage N., Yewale S., Yarish A., Lanham D., Bulas D., et al. (2018). In vivo placental MRI shape and textural features predict fetal growth restriction and postnatal outcome. J. Magn. Reson. Imaging. 47 (2), 449–458. doi:10.1002/jmri.25806
Dall'Asta A., Ghi T., Rizzo G., Cancemi A., Aloisio F., Arduini D., et al. (2019). Cerebroplacental ratio assessment in early labor in uncomplicated term pregnancy and prediction of adverse perinatal outcome: Prospective multicenter study. Ultrasound Obstet. Gynecol. 53 (4), 481–487. doi:10.1002/uog.19113
Dall'Asta A., Stampalija T., Mecacci F., Minopoli M., Schera G. B. L., Cagninelli G., et al. (2022). Ultrasound prediction of adverse perinatal outcome at diagnosis of late-onset fetal growth restriction. Ultrasound Obstet. Gynecol. 59 (3), 342–349. doi:10.1002/uog.23714
Dall’Asta A., Brunelli V., Prefumo F., Frusca T., Lees C. C. (2017). Early onset fetal growth restriction. Matern. Health Neonatol. Perinatol. 3, 2. doi:10.1186/s40748-016-0041-x
Damhuis S. E., Ganzevoort W., Gordijn S. J. (2021). Abnormal fetal growth: Small for gestational age, fetal growth restriction, large for gestational age: Definitions and epidemiology. Obstet. Gynecol. Clin. North Am. 48 (2), 267–279. doi:10.1016/j.ogc.2021.02.002
Dawes G. S., Fox H. E., Leduc B. M., Liggins G. C., Richards R. T. (1972). Respiratory movements and rapid eye movement sleep in the foetal lamb. J. Physiol. 220 (1), 119–143. doi:10.1113/jphysiol.1972.sp009698
Dawes G. S., Mott J. C., Shelley H. J. (1959). The importance of cardiac glycogen for the maintenance of life in foetal lambs and newborn animals during anoxia. J. Physiol. 146 (3), 516–538. doi:10.1113/jphysiol.1959.sp006208
de Vries J. I., Visser G. H., Mulder E. J., Prechtl H. F. (1987). Diurnal and other variations in fetal movement and heart rate patterns at 20-22 weeks. Early Hum. Dev. 15 (6), 333–348. doi:10.1016/0378-3782(87)90029-6
Delnord M., Zeitlin J. (2019). Epidemiology of late preterm and early term births – an international perspective. Semin. Fetal Neonatal Med. 24 (1), 3–10. doi:10.1016/j.siny.2018.09.001
Deter R. L., Lee W., Dicker P., Tully E. C., Cody F., Malone F. D., et al. (2021). Third-trimester growth diversity in small fetuses classified as appropriate-for-gestational age or small-for-gestational age at birth. Ultrasound Obstet. Gynecol. 58 (6), 882–891. doi:10.1002/uog.23688
Di Pasquo E., Ghi T., Dall'Asta A., Angeli L., Ciavarella S., Armano G., et al. (2021). Hemodynamic findings in normotensive women with small-for-gestational-age and growth-restricted fetuses. Acta Obstet. Gynecol. Scand. 100 (5), 876–883. doi:10.1111/aogs.14026
Dimasi C. G., Lazniewska J., Plush S. E., Saini B. S., Holman S. L., Cho S. K. S., et al. (2021). Redox ratio in the left ventricle of the growth restricted fetus is positively correlated with cardiac output. J. Biophot. 14 (12), e202100157. doi:10.1002/jbio.202100157
Drake R. R., Louey S., Thornburg K. L. (2022). Intrauterine growth restriction elevates circulating acylcarnitines and suppresses fatty acid metabolism genes in the fetal sheep heart. J. Physiol. 600 (3), 655–670. doi:10.1113/jp281415
Dubucs C., Groussolles M., Ousselin J., Sartor A., Van Acker N., Vayssière C., et al. (2022). Severe placental lesions due to maternal SARS-CoV-2 infection associated to intrauterine fetal death. Hum. Pathol. 121, 46–55. doi:10.1016/j.humpath.2021.12.012
Ducsay C. A., Goyal R., Pearce W. J., Wilson S., Hu X. Q., Zhang L. (2018). Gestational hypoxia and developmental plasticity. Physiol. Rev. 98 (3), 1241–1334. doi:10.1152/physrev.00043.2017
Dudink I., Hüppi P. S., Sizonenko S. V., Castillo-Melendez M., Sutherland A. E., Allison B. J., et al. (2022). Altered trajectory of neurodevelopment associated with fetal growth restriction. Exp. Neurol. 347, 113885. doi:10.1016/j.expneurol.2021.113885
Ego A., Monier I., Skaare K., Zeitlin J. (2020). Antenatal detection of fetal growth restriction and risk of stillbirth: Population-based case-control study. Ultrasound Obstet. Gynecol. 55 (5), 613–620. doi:10.1002/uog.20414
Esposito F. G., Tagliaferri S., Giudicepietro A., Giuliano N., Maruotti G. M., Saccone G., et al. (2019). Fetal heart rate monitoring and neonatal outcome in a population of early- and late-onset intrauterine growth restriction. J. Obstet. Gynaecol. Res. 45 (7), 1343–1351. doi:10.1111/jog.13981
Ferrazzi E., Bulfamante G., Mezzopane R., Barbera A., Ghidini A., Pardi G. (1999). Uterine Doppler velocimetry and placental hypoxic-ischemic lesion in pregnancies with fetal intrauterine growth restriction. Placenta 20 (5-6), 389–394. doi:10.1053/plac.1999.0395
Figueras F., Caradeux J., Crispi F., Eixarch E., Peguero A., Gratacos E. (2018). Diagnosis and surveillance of late-onset fetal growth restriction. Am. J. Obstet. Gynecol. 218 (2), S790S790–S802.e1. doi:10.1016/j.ajog.2017.12.003
Figueras F., Gratacós E. (2014). Update on the diagnosis and classification of fetal growth restriction and proposal of a stage-based management protocol. Fetal diagn. Ther. 36 (2), 86–98. doi:10.1159/000357592
Figueras F., Oros D., Cruz-Martinez R., Padilla N., Hernandez-Andrade E., Botet F., et al. (2009). Neurobehavior in term, small-for-gestational age infants with normal placental function. Pediatrics 124 (5), e934–941. doi:10.1542/peds.2008-3346
Frasch M. G., Lobmaier S. M., Stampalija T., Desplats P., Pallarés M. E., Pastor V., et al. (2018). Non-invasive biomarkers of fetal brain development reflecting prenatal stress: An integrative multi-scale multi-species perspective on data collection and analysis. Neurosci. Biobehav. Rev. 117, 165–183. doi:10.1016/j.neubiorev.2018.05.026
Fu J., Olofsson P. (2006). Restrained cerebral hyperperfusion in response to superimposed acute hypoxemia in growth-restricted human fetuses with established brain-sparing blood flow. Early Hum. Dev. 82 (3), 211–216. doi:10.1016/j.earlhumdev.2005.09.009
Fung A. M., Wilson D. L., Lappas M., Howard M., Barnes M., O'Donoghue F., et al. (2013). Effects of maternal obstructive sleep apnoea on fetal growth: A prospective cohort study. PloS One 8 (7), e68057. doi:10.1371/journal.pone.0068057
Fung C., Zinkhan E. (2021). Short- and long-term implications of small for gestational age. Obstet. Gynecol. Clin. North Am. 48 (2), 311–323. doi:10.1016/j.ogc.2021.02.004
Ganzevoort W., Thornton J. G., Marlow N., Thilaganathan B., Arabin B., Prefumo F., et al. (2020). Comparative analysis of 2-year outcomes in GRIT and TRUFFLE trials. Ultrasound Obstet. Gynecol. 55 (1), 68–74. doi:10.1002/uog.20354
Garcia-Manau P., Mendoza M., Bonacina E., Garrido-Gimenez C., Fernandez-Oliva A., Zanini J., et al. (2021). Soluble fms-like tyrosine kinase to placental growth factor ratio in different stages of early-onset fetal growth restriction and small for gestational age. Acta Obstet. Gynecol. Scand. 100 (1), 119–128. doi:10.1111/aogs.13978
Gardner D. S., Giussani D. A. (2003). Enhanced umbilical blood flow during acute hypoxemia after chronic umbilical cord compression: A role for nitric oxide. Circulation 108 (3), 331–335. doi:10.1161/01.Cir.0000080323.40820.A1
Gardosi J., Francis A., Turner S., Williams M. (2018). Customized growth charts: Rationale, validation and clinical benefits. Am. J. Obstet. Gynecol. 218 (2), S609–S618. doi:10.1016/j.ajog.2017.12.011
Genc S., Ozer H., Emeklioglu C. N., Cingillioglu B., Sahin O., Akturk E., et al. (2022). Relationship between extreme values of first trimester maternal pregnancy associated plasma Protein-A, free-β-human chorionic gonadotropin, nuchal translucency and adverse pregnancy outcomes. Taiwan. J. Obstet. Gynecol. 61 (3), 433–440. doi:10.1016/j.tjog.2022.02.043
Giussani D. A. (2016). The fetal brain sparing response to hypoxia: Physiological mechanisms. J. Physiol. 594 (5), 1215–1230. doi:10.1113/JP271099
Gordijn S. J., Beune I. M., Thilaganathan B., Papageorghiou A., Baschat A. A., Baker P. N., et al. (2016). Consensus definition of fetal growth restriction: A Delphi procedure. Ultrasound Obstet. Gynecol. 48 (3), 333–339. doi:10.1002/uog.15884
Gordon A. F., Raynes-Greenow C., Bond D. R., Morris J. F., Rawlinson W. F., Jeffery H. F. (2015). Sleep position, fetal growth restriction, and late-pregnancy stillbirth: The sydney stillbirth study. Obstet. Gynecol. 125 (2), 347–355. doi:10.1097/AOG.0000000000000627
Graatsma E. M., Mulder E. J. H., Vasak B., Lobmaier S. M., Pildner von Steinburg S., Schneider K. T. M., et al. (2012). Average acceleration and deceleration capacity of fetal heart rate in normal pregnancy and in pregnancies complicated by fetal growth restriction. J. Matern. Fetal. Neonatal Med.the Int. Soc. Perinat. Obstetricians 25 (12), 2517–2522. doi:10.3109/14767058.2012.704446
Haws R. A., Yakoob M. Y., Soomro T., Menezes E. V., Darmstadt G. L., Bhutta Z. A. (2009). Reducing stillbirths: Screening and monitoring during pregnancy and labour. BMC Pregnancy Childbirth 9 (1), S5. doi:10.1186/1471-2393-9-s1-s5)
Heazell A. E. P., Budd J., Li M., Cronin R., Bradford B., McCowan L. M. E., et al. (2018a). Alterations in maternally perceived fetal movement and their association with late stillbirth: Findings from the midland and north of england stillbirth case–control study. BMJ Open 8 (7), e020031. doi:10.1136/bmjopen-2017-020031
Heazell A. E. P., Frøen J. F. (2008). Methods of fetal movement counting and the detection of fetal compromise. J. Obstet. Gynaecol. 28 (2), 147–154. doi:10.1080/01443610801912618
Heazell A. E. P., Stacey T., O'Brien L. M., Mitchell E. A., Warland J. (2018b). Excessive fetal movements are a sign of fetal compromise which merits further examination. Med. Hypotheses 111, 19–23. doi:10.1016/j.mehy.2017.12.024
Heazell A. E. P., Worton S. A., Higgins L. E., Ingram E., Johnstone E. D., Jones R. L., et al. (2015). IFPA Gábor than Award Lecture: Recognition of placental failure is key to saving babies' lives. Placenta 36, S20–S28. doi:10.1016/j.placenta.2014.12.017
Henderson J., Roberts T., Sikorski J., Wilson J., Clement S. (2000). An economic evaluation comparing two schedules of antenatal visits. J. Health Serv. Res. Policy 5 (2), 69–75. doi:10.1177/135581960000500203
Henrichs J., Verfaille V., Jellema P., Viester L., Pajkrt E., Wilschut J., et al. (2019). Effectiveness of routine third trimester ultrasonography to reduce adverse perinatal outcomes in low risk pregnancy (the IRIS study): Nationwide, pragmatic, multicentre, stepped wedge cluster randomised trial. BMJ Clin. Res. ed.) 367, l5517. doi:10.1136/bmj.l5517
Herrera E. A., Rojas R. T., Krause B. J., Ebensperger G., Reyes R. V., Giussani D. A., et al. (2016). Cardiovascular function in term fetal sheep conceived, gestated and studied in the hypobaric hypoxia of the Andean altiplano. J. Physiol. 594 (5), 1231–1245. doi:10.1113/JP271110
Hiersch L., Melamed N. (2018). Fetal growth velocity and body proportion in the assessment of growth. Am. J. Obstet. Gynecol. 218 (2), S700S700–S711.e1. doi:10.1016/j.ajog.2017.12.014
Ho A., Chappell L. C., Story L., Al-Adnani M., Egloff A., Routledge E., et al. (2022). Visual assessment of the placenta in antenatal magnetic resonance imaging across gestation in normal and compromised pregnancies: Observations from a large cohort study. Placenta 117, 29–38. doi:10.1016/j.placenta.2021.10.006
Ho A., Hutter J., Slator P., Jackson L., Seed P. T., McCabe L., et al. (2021). Placental magnetic resonance imaging in chronic hypertension: A case-control study. Placenta 104, 138–145. doi:10.1016/j.placenta.2020.12.006
Hoyer D., Schmidt A., Gustafson K. M., Lobmaier S. M., Lakhno I., van Leeuwen P., et al. (2019). Heart rate variability categories of fluctuation amplitude and complexity: Diagnostic markers of fetal development and its disturbances. Physiol. Meas. 40 (6), 064002. doi:10.1088/1361-6579/ab205f
Hoyer D., Żebrowski J., Cysarz D., Gonçalves H., Pytlik A., Amorim-Costa C., et al. (2017). Monitoring fetal maturation-objectives, techniques and indices of autonomic function. Physiol. Meas. 38 (5), R61–R88. doi:10.1088/1361-6579/aa5fca
Hromadnikova I., Kotlabova K., Krofta L. (2022). First-trimester screening for fetal growth restriction and small-for-gestational-age pregnancies without preeclampsia using cardiovascular disease-associated MicroRNA biomarkers. Biomedicines 10 (3), 718. doi:10.3390/biomedicines10030718
Huhn E. A., Lobmaier S., Fischer T., Schneider R., Bauer A., Schneider K. T., et al. (2011). New computerized fetal heart rate analysis for surveillance of intrauterine growth restriction. Prenat. Diagn. 31 (5), 509–514. doi:10.1002/pd.2728
Humphries A., Mirjalili S. A., Tarr G. P., Thompson J. M. D., Stone P. (2018). The effect of supine positioning on maternal hemodynamics during late pregnancy. J. Matern. Fetal. Neonatal Med. 0 (0), 3923–3930. doi:10.1080/14767058.2018.1478958
Ingram E., Morris D., Naish J., Myers J., Johnstone E. (2017). MR imaging measurements of altered placental oxygenation in pregnancies complicated by fetal growth restriction. Radiology 285 (3), 953–960. doi:10.1148/radiol.2017162385
Jacquier M., Salomon L. J. (2021). Multi-compartment MRI as a promising tool for measurement of placental perfusion and oxygenation in early-onset fetal growth restriction. Bjog 128 (2), 346. doi:10.1111/1471-0528.16459
Kalafat E., Khalil A. (2018). Clinical significance of cerebroplacental ratio. Curr. Opin. Obstet. Gynecol. 30 (6), 344–354. doi:10.1097/gco.0000000000000490
Kalafat E., Ozturk E., Sivanathan J., Thilaganathan B., Khalil A. (2019). Longitudinal change in cerebroplacental ratio in small-for-gestational-age fetuses and risk of stillbirth. Ultrasound Obstet. Gynecol. 54 (4), 492–499. doi:10.1002/uog.20193
Kametas N. A., Nzelu D., Nicolaides K. H. (2022). Chronic hypertension and superimposed preeclampsia: Screening and diagnosis. Am. J. Obstet. Gynecol. 226 (2S), S1182–S1195. doi:10.1016/j.ajog.2020.11.029
Kapaya H., Broughton Pipkin F., Hayes-Gill B., Loughna P. V. (2016). Circadian changes and sex-related differences in fetal heart rate parameters. Matern. Health Neonatol. Perinatol. 2 (1), 9. doi:10.1186/s40748-016-0037-6
Kapaya H., Jacques R., Anumba D. (2018). Comparison of diurnal variations, gestational age and gender related differences in fetal heart rate (FHR) parameters between appropriate-for-gestational-age (AGA) and small-for-gestational-age (SGA) fetuses in the home environment. PloS One 13 (3), e0193908. doi:10.1371/journal.pone.0193908
Keen A. E., Frasch M. G., Sheehan M. A., Matushewski B. J., Richardson B. S. (2011). Electrocortical activity in the near-term ovine fetus: Automated analysis using amplitude frequency components. Brain Res. 1402, 30–37. doi:10.1016/j.brainres.2011.05.044
Kennedy L. M., Tong S., Robinson A. J., Hiscock R. J., Hui L., Dane K. M., et al. (2020). Reduced growth velocity from the mid-trimester is associated with placental insufficiency in fetuses born at a normal birthweight. BMC Med. 18 (1), 395. doi:10.1186/s12916-020-01869-3
Khalil A. A., Morales-Rosello J., Morlando M., Hannan H., Bhide A., Papageorghiou A., et al. (2015). Is fetal cerebroplacental ratio an independent predictor of intrapartum fetal compromise and neonatal unit admission? Am. J. Obstet. Gynecol. 213 (1), 54. doi:10.1016/j.ajog.2014.10.024
Khalil A., Gordijn S. J., Beune I. M., Wynia K., Ganzevoort W., Figueras F., et al. (2019). Essential variables for reporting research studies on fetal growth restriction: A Delphi consensus. Ultrasound Obstet. Gynecol. 53 (5), 609–614. doi:10.1002/uog.19196
Khalil A., Thilaganathan B. (2017). Role of uteroplacental and fetal Doppler in identifying fetal growth restriction at term. Best. Pract. Res. Clin. Obstet. Gynaecol. 38, 38–47. doi:10.1016/j.bpobgyn.2016.09.003
Khatibi T., Hanifi E., Sepehri M. M., Allahqoli L. (2021). Proposing a machine-learning based method to predict stillbirth before and during delivery and ranking the features: Nationwide retrospective cross-sectional study. BMC Pregnancy Childbirth 21, 202. doi:10.1186/s12884-021-03658-z
Kingdom J. C., Audette M. C., Hobson S. R., Windrim R. C., Morgen E. (2018). A placenta clinic approach to the diagnosis and management of fetal growth restriction. Am. J. Obstet. Gynecol. 218 (2), S803–S817. doi:10.1016/j.ajog.2017.11.575
Kinoshita M., Thuring A., Morsing E., Maršál K. (2021). Extent of absent end-diastolic flow in umbilical artery and outcome of pregnancy. Ultrasound Obstet. Gynecol. 58 (3), 369–376. doi:10.1002/uog.23541
Kiserud T., Jauniaux E., West D., Ozturk O., Hanson M. A. (2001). Circulatory responses to maternal hyperoxaemia and hypoxaemia assessed non-invasively in fetal sheep at 0.3-0.5 gestation in acute experiments. Bjog 108 (4), 359–364. doi:10.1111/j.1471-0528.2001.00096.x
Kiserud T., Piaggio G., Carroli G., Widmer M., Carvalho J., Neerup Jensen L., et al. (2017). The world health organization fetal growth charts: A multinational longitudinal study of ultrasound biometric measurements and estimated fetal weight. PLoS Med. 14 (1), e1002220. doi:10.1371/journal.pmed.1002220
Kneitel A. W., Treadwell M. C., O’Brien L. M. (2018). Effects of maternal obstructive sleep apnea on fetal growth: A case-control study. J. Perinatol. 38 (8), 982–988. doi:10.1038/s41372-018-0127-6
Kovo M., Schreiber L., Ben-Haroush A., Wand S., Golan A., Bar J. (2010). Placental vascular lesion differences in pregnancy-induced hypertension and normotensive fetal growth restriction. Am. J. Obstet. Gynecol. 202 (6), 561.e1–561.e5. doi:10.1016/j.ajog.2010.01.012
Lausman A., Kingdom J. (2021). How and when to recommend delivery of a growth-restricted fetus: A review. Best. Pract. Res. Clin. Obstet. Gynaecol. 77, 119–128. doi:10.1016/j.bpobgyn.2021.09.006
Lear C. A., Davidson J. O., Mackay G. R., Drury P. P., Galinsky R., Quaedackers J. S., et al. (2018). Antenatal dexamethasone before asphyxia promotes cystic neural injury in preterm fetal sheep by inducing hyperglycemia. J. Cereb. Blood Flow. Metab. 38 (4), 706–718. doi:10.1177/0271678X17703124
Lear C. A., Galinsky R., Wassink G., Yamaguchi K., Davidson J. O., Westgate J. A., et al. (2016). The myths and physiology surrounding intrapartum decelerations: The critical role of the peripheral chemoreflex. J. Physiol. 594 (17), 4711–4725. doi:10.1113/JP271205
Lee S. J., Hatran D. P., Tomimatsu T., Pena J. P., McAuley G., Longo L. D. (2009). Fetal cerebral blood flow, electrocorticographic activity, and oxygenation: Responses to acute hypoxia. J. Physiol. 587 (9), 2033–2047. doi:10.1113/jphysiol.2009.166983
Lees C. C., Marlow N., van Wassenaer-Leemhuis A., Arabin B., Bilardo C. M., Brezinka C., et al. (2015). 2 year neurodevelopmental and intermediate perinatal outcomes in infants with very preterm fetal growth restriction (TRUFFLE): A randomised trial. Lancet 385 (9983), 2162–2172. doi:10.1016/S0140-6736(14)62049-3
Lees C. C., Romero R., Stampalija T., Dall'Asta A., DeVore G. A., Prefumo F., et al. (2022). Clinical opinion: The diagnosis and management of suspected fetal growth restriction: An evidence-based approach. Am. J. Obstet. Gynecol. 226 (3), 366–378. doi:10.1016/j.ajog.2021.11.1357
Lees C., Marlow N., Arabin B., Bilardo C. M., Brezinka C., Derks J. B., et al. (2013). Perinatal morbidity and mortality in early-onset fetal growth restriction: Cohort outcomes of the trial of randomized umbilical and fetal flow in Europe (TRUFFLE). Ultrasound Obstet. Gynecol. 42 (4), 400–408. doi:10.1002/uog.13190
Liu X.-L., Feng J., Huang C.-T., Mei Y.-J., Xu Y.-K. (2021). Use of intravoxel incoherent motion MRI to assess placental perfusion in normal and Fetal Growth Restricted pregnancies on their third trimester. Placenta 118, 10–15. doi:10.1016/j.placenta.2021.12.019
Llanos A. J., Green J. R., Creasy R. K., Rudolph A. M. (1980). Increased heart rate response to parasympathetic and beta adrenergic blockade in growth-retarded fetal lambs. Am. J. Obstet. Gynecol. 136 (6), 808–813. doi:10.1016/0002-9378(80)90460-3
Longo S., Borghesi A., Tzialla C., Stronati M. (2014). IUGR and infections. Early Hum. Dev. 90, S42–S44. doi:10.1016/S0378-3782(14)70014-3
Louey S., Jonker S. S., Giraud G. D., Thornburg K. L. (2007). Placental insufficiency decreases cell cycle activity and terminal maturation in fetal sheep cardiomyocytes. J. Physiol. 580 (2), 639–648. doi:10.1113/jphysiol.2006.122200
Lunshof S., Boer K., Wolf H., van Hoffen G., Bayram N., Mirmiran M. (1998). Fetal and maternal diurnal rhythms during the third trimester of normal pregnancy: Outcomes of computerized analysis of continuous twenty-four-hour fetal heart rate recordings. Am. J. Obstet. Gynecol. 178 (2), 247–254. doi:10.1016/s0002-9378(98)80008-2
Malhotra A., Allison B. J., Castillo-Melendez M., Jenkin G., Polglase G. R., Miller S. L. (2019). Neonatal morbidities of fetal growth restriction: Pathophysiology and impact. Front. Endocrinol. 10, 55. doi:10.3389/fendo.2019.00055
Maréchal L., Sicotte B., Caron V., Brochu M., Tremblay A. (2021). Fetal cardiac lipid sensing triggers an early and sex-related metabolic energy switch in intrauterine growth restriction. J. Clin. Endocrinol. Metab. 106 (11), 3295–3311. doi:10.1210/clinem/dgab496
Masoumy E. P., Sawyer A. A., Sharma S., Patel J. A., Gordon P. M. K., Regnault T. R. H., et al. (2018). The lifelong impact of fetal growth restriction on cardiac development. Pediatr. Res. 84 (4), 537–544. doi:10.1038/s41390-018-0069-x
McCowan L. M. E., Thompson J. M. D., Taylor R. S., Baker P. N., North R. A., Poston L., et al. (2017). Prediction of small for gestational age infants in healthy nulliparous women using clinical and ultrasound risk factors combined with early pregnancy biomarkers. PLOS ONE 12 (1), e0169311. doi:10.1371/journal.pone.0169311
McCowan L. M., Figueras F., Anderson N. H. (2018). Evidence-based national guidelines for the management of suspected fetal growth restriction: Comparison, consensus, and controversy. Am. J. Obstet. Gynecol. 218 (2), S855–S868. doi:10.1016/j.ajog.2017.12.004
Mellor D. J., Diesch T. J., Gunn A. J., Bennet L. (2005). The importance of ‘awareness’ for understanding fetal pain. Brain Res. Brain Res. Rev. 49 (3), 455–471. doi:10.1016/j.brainresrev.2005.01.006
Mendoza M., Serrano B., Bonacina E., Capote S., Garcia-Manau P., Regincós L., et al. (2022). Diagnostic accuracy of the Gaussian first-trimester combined screening for pre-eclampsia to predict small-for-gestational-age neonates. Int. J. Gynaecol. Obstet. 156 (2), 322–330. doi:10.1002/ijgo.13673
Mifsud W., Sebire N. J. (2014). Placental pathology in early-onset and late-onset fetal growth restriction. Fetal diagn. Ther. 36 (2), 117–128. doi:10.1159/000359969
Miller S. L., Huppi P. S., Mallard C. (2016). The consequences of fetal growth restriction on brain structure and neurodevelopmental outcome. J. Physiol. 594 (4), 807–823. doi:10.1113/JP271402
Miranda J., Rodriguez-Lopez M., Triunfo S., Sairanen M., Kouru H., Parra-Saavedra M., et al. (2017). Prediction of fetal growth restriction using estimated fetal weight vs a combined screening model in the third trimester. Ultrasound Obstet. Gynecol. 50 (5), 603–611. doi:10.1002/uog.17393
Mitchell E. K., Louey S., Cock M. L., Harding R., Black M. J. (2004). Nephron endowment and filtration surface area in the kidney after growth restriction of fetal sheep. Pediatr. Res. 55 (5), 769–773. doi:10.1203/01.Pdr.0000120681.61201.B4
Molina L. C. G., Odibo L., Zientara S., Običan S. G., Rodriguez A., Stout M., et al. (2020). Validation of Delphi procedure consensus criteria for defining fetal growth restriction. Ultrasound Obstet. Gynecol. 56 (1), 61–66. doi:10.1002/uog.20854
Monteith C., Flood K., Pinnamaneni R., Levine T. A., Alderdice F. A., Unterscheider J., et al. (2019). An abnormal cerebroplacental ratio (CPR) is predictive of early childhood delayed neurodevelopment in the setting of fetal growth restriction. Am. J. Obstet. Gynecol. 221 (3), 273.e1–273273.e9273. e279. doi:10.1016/j.ajog.2019.06.026
Morales-Roselló J., Khalil A., Fornés-Ferrer V., Alberola-Rubio J., Hervas-Marín D., Peralta Llorens N., et al. (2018). Progression of Doppler changes in early-onset small for gestational age fetuses. How frequent are the different progression sequences? J. Matern. Fetal. Neonatal Med. 31 (8), 1000–1008. doi:10.1080/14767058.2017.1304910
Morris R. K., Bilagi A., Devani P., Kilby M. D. (2017). Association of serum PAPP-A levels in first trimester with small for gestational age and adverse pregnancy outcomes: Systematic review and meta-analysis. Prenat. Diagn. 37 (3), 253–265. doi:10.1002/pd.5001
Murphy C. N., Cluver C. A., Walker S. P., Keenan E., Hastie R., MacDonald T. M., et al. (2022). Circulating SPINT1 is reduced in a preeclamptic cohort with Co-existing fetal growth restriction. J. Clin. Med. 11 (4), 901. doi:10.3390/jcm11040901
Murphy C. N., Walker S. P., MacDonald T. M., Keenan E., Hannan N. J., Wlodek M. E., et al. (2021). Elevated circulating and placental SPINT2 is associated with placental dysfunction. Int. J. Mol. Sci. 22 (14), 7467. doi:10.3390/ijms22147467
Murray H. (2017). Antenatal foetal heart monitoring. Best. Pract. Res. Clin. Obstet. Gynaecol. 38, 2–11. doi:10.1016/j.bpobgyn.2016.10.008
Nowakowska B. A., Pankiewicz K., Nowacka U., Niemiec M., Kozłowski S., Issat T. (2021). Genetic background of fetal growth restriction. Int. J. Mol. Sci. 23 (1), 36. doi:10.3390/ijms23010036
Oluklu D., Beser D. M., Hendem D. U., Kara O., Yazihan N., Sahin D. (2022). Maternal serum midkine level in fetal growth restriction: A case-control study. J. Perinat. Med. 0. doi:10.1515/jpm-2022-0019
Pacora P., Romero R., Jaiman S., Erez O., Bhatti G., Panaitescu B., et al. (2019). Mechanisms of death in structurally normal stillbirths. J. Perinat. Med. 47 (2), 222–240. doi:10.1515/jpm-2018-0216
Pacora P., Romero R., Jung E., Gudicha D. W., Hernandez-Andrade E., Musilova I., et al. (2021). Reduced fetal growth velocity precedes antepartum fetal death. Ultrasound Obstet. Gynecol. 57 (6), 942–952. doi:10.1002/uog.23111
Pagani G., D'Antonio F., Khalil A., Akolekar R., Papageorghiou A., Bhide A., et al. (2014). Association between reduced fetal movements at term and abnormal uterine artery Doppler indices. Ultrasound Obstet. Gynecol. 43 (5), 548–552. doi:10.1002/uog.13220
Paoletti D., Smyth L., Westerway S., Hyett J., Mogra R., Haslett S., et al. (2021). A survey of current practice in reporting third trimester fetal biometry and Doppler in Australia and New Zealand. Australas. J. Ultrasound Med. 24 (4), 225–237. doi:10.1002/ajum.12282
Paules C., Youssef L., Miranda J., Crovetto F., Estanyol J. M., Fernandez G., et al. (2020). Maternal proteomic profiling reveals alterations in lipid metabolism in late-onset fetal growth restriction. Sci. Rep. 10 (1), 21033. doi:10.1038/s41598-020-78207-3
Pecks U., Rath W., Maass N., Berger B., Lueg I., Farrokh A., et al. (2016). Fetal gender and gestational age differentially affect PCSK9 levels in intrauterine growth restriction. Lipids Health Dis. 15 (1), 193. doi:10.1186/s12944-016-0365-6
Perry H., Lehmann H., Mantovani E., Thilaganathan B., Khalil A. (2020). Are maternal hemodynamic indices markers of fetal growth restriction in pregnancies with a small-for-gestational-age fetus? Ultrasound Obstet. Gynecol. 55 (2), 210–216. doi:10.1002/uog.20419
Pini N., Lucchini M., Esposito G., Tagliaferri S., Campanile M., Magenes G., et al. (2021). A machine learning approach to monitor the emergence of late intrauterine growth restriction. Front. Artif. Intell. 4, 622616. doi:10.3389/frai.2021.622616
Pulgar V. M., Hong J. K., Jessup J. A., Massmann A. G., Diz D. I., Figueroa J. P. (2009). Mild chronic hypoxemia modifies expression of brain stem angiotensin peptide receptors and reflex responses in fetal sheep. Am. J. Physiol. Regul. Integr. Comp. Physiol. 297 (2), R446–R452. doi:10.1152/ajpregu.00023.2009
Pulgar V. M., Zhang J., Massmann G. A., Figueroa J. P. (2006). Prolonged mild hypoxia alters fetal sheep electrocorticogram activity. J. Soc. Gynecol. Investig. 13 (6), 404–411. doi:10.1016/j.jsgi.2006.05.007
Richardson B. S., Caetano H., Homan J., Carmichael L. (1994). Regional brain blood flow in the ovine fetus during transition to the low-voltage electrocortical state. Brain Res. Dev. Brain Res. 81 (1), 10–16. doi:10.1016/0165-3806(94)90063-9
Robertson N. T., Turner J. M., Kumar S. (2019). Pathophysiological changes associated with sleep disordered breathing and supine sleep position in pregnancy. Sleep. Med. Rev. 46, 1–8. doi:10.1016/j.smrv.2019.04.006
Robinson J. S., Jones C. T., Kingston E. J. (1983). Studies on experimental growth retardation in sheep. The effects of maternal hypoxaemia. J. Dev. Physiol. 5 (2), 89–100.
Roma E., Arnau A., Berdala R., Bergos C., Montesinos J., Figueras F. (2015). Ultrasound screening for fetal growth restriction at 36 vs 32 weeks' gestation: A randomized trial (ROUTE). Ultrasound Obstet. Gynecol. 46 (4), 391–397. doi:10.1002/uog.14915
Ryo E., Kamata H., Seto M., Morita M., Nagaya Y., Nishihara K., et al. (2018). Reference values for a fetal movement acceleration measurement recorder to count fetal movements. Pediatr. Res. 83 (5), 961–968. doi:10.1038/pr.2017.328
Saini B. S., Darby J. R. T., Marini D., Portnoy S., Lock M. C., Yin Soo J., et al. (2021). An MRI approach to assess placental function in healthy humans and sheep. J. Physiol. 599 (10), 2573–2602. doi:10.1113/JP281002
Sandoval Karamian A. G., Wusthoff C. J. (2021). Current and future uses of continuous EEG in the NICU. Front. Pediatr. 9, 768670. doi:10.3389/fped.2021.768670
Schiffer V., van Haren A., De Cubber L., Bons J., Coumans A., van Kuijk S. M., et al. (2021). Ultrasound evaluation of the placenta in healthy and placental syndrome pregnancies: A systematic review. Eur. J. Obstet. Gynecol. Reprod. Biol. 262, 45–56. doi:10.1016/j.ejogrb.2021.04.042
Sharma D., Shastri S., Sharma P. (2016). Intrauterine growth restriction: Antenatal and postnatal aspects. Clin. Med. Insights. Pediatr. 10, 67–83. doi:10.4137/CMPed.S40070
Sharony R., Sharon-Weiner M., Kidron D., Sukenik-Halevy R., Biron-Shental T., Manor M., et al. (2018). The association between maternal serum first trimester free βhCG, second trimester intact hCG levels and foetal growth restriction and preeclampsia. J. Obstet. Gynaecol. 38 (3), 363–366. doi:10.1080/01443615.2017.1340441
Shaw C. J., Allison B. J., Itani N., Botting K. J., Niu Y., Lees C. C., et al. (2018). Altered autonomic control of heart rate variability in the chronically hypoxic fetus. J. Physiol. 596 (23), 6105–6119. doi:10.1113/JP275659
Shelley H. J. (1964). Carbohydrate reserves in the newborn infant. Br. Med. J. 1 (5378), 273–275. doi:10.1136/bmj.1.5378.273
Signorini M. G., Magenes G., Cerutti S., Arduini D. (2003). Linear and nonlinear parameters for the analysisof fetal heart rate signal from cardiotocographic recordings. IEEE Trans. Biomed. Eng. 50 (3), 365–374. doi:10.1109/TBME.2003.808824
Signorini M. G., Pini N., Malovini A., Bellazzi R., Magenes G. (2020). Integrating machine learning techniques and physiology based heart rate features for antepartum fetal monitoring. Comput. Methods Programs Biomed. 185, 105015. doi:10.1016/j.cmpb.2019.105015
Sinding M., Peters D. A., Frokjaer J. B., Christiansen O. B., Petersen A., Uldbjerg N., et al. (2016). Placental magnetic resonance imaging T2* measurements in normal pregnancies and in those complicated by fetal growth restriction. Ultrasound Obstet. Gynecol. 47 (6), 748–754. doi:10.1002/uog.14917
Smith G. C., Moraitis A. A., Wastlund D., Thornton J. G., Papageorghiou A., Sanders J., et al. (2021). Universal late pregnancy ultrasound screening to predict adverse outcomes in nulliparous women: A systematic review and cost-effectiveness analysis. Health Technol. Assess. 25 (15), 1–190. doi:10.3310/hta25150
Smith G. C. S. (2021). Developing novel tests to screen for fetal growth restriction. Trends Mol. Med. 27 (8), 743–752. doi:10.1016/j.molmed.2021.05.006
Sovio U., Goulding N., McBride N., Cook E., Gaccioli F., Charnock-Jones D. S., et al. (2020). A maternal serum metabolite ratio predicts fetal growth restriction at term. Nat. Med. 26 (3), 348–353. doi:10.1038/s41591-020-0804-9
Sovio U., Smith G. C. S. (2018). The effect of customization and use of a fetal growth standard on the association between birthweight percentile and adverse perinatal outcome. Am. J. Obstet. Gynecol. 218 (2), S738–S744. doi:10.1016/j.ajog.2017.11.563
Sriram B., Mencer M. A., McKelvey S., Siegel E. R., Vairavan S., Wilson J. D., et al. (2013). Differences in the sleep states of IUGR and low-risk fetuses: An MCG study. Early Hum. Dev. 89 (10), 815–819. doi:10.1016/j.earlhumdev.2013.07.002
Stampalija T., Casati D., Monasta L., Sassi R., Rivolta M. W., Muggiasca M. L., et al. (2016). Brain sparing effect in growth-restricted fetuses is associated with decreased cardiac acceleration and deceleration capacities: A case-control study. BJOG Int. J. obstetrics Gynaecol. 123 (12), 1947–1954. doi:10.1111/1471-0528.13607
Stampalija T., Casati D., Montico M., Sassi R., Rivolta M. W., Maggi V., et al. (2015). Parameters influence on acceleration and deceleration capacity based on trans-abdominal ECG in early fetal growth restriction at different gestational age epochs. Eur. J. Obstet. Gynecol. Reprod. Biol. 188, 104–112. doi:10.1016/j.ejogrb.2015.03.003
Stone P. R., Burgess W., McIntyre J. P. R., Gunn A. J., Lear C. A., Bennet L., et al. (2017). Effect of maternal position on fetal behavioural state and heart rate variability in healthy late gestation pregnancy. J. Physiol. 595 (4), 1213–1221. doi:10.1113/JP273201
Stroux L., Redman C. W., Georgieva A., Payne S. J., Clifford G. D. (2017). Doppler-based fetal heart rate analysis markers for the detection of early intrauterine growth restriction. Acta Obstet. Gynecol. Scand. 96 (11), 1322–1329. doi:10.1111/aogs.13228
Sumiyoshi K., Kawagoe Y., Ohhashi M., Furukawa S., Sameshima H., Ikenoue T. (2020). Delayed rhythm formation of normal-structured, growth-restricted fetuses using fetal heart rate monitoring patterns. J. Obstet. Gynaecol. Res. 46 (8), 1342–1348. doi:10.1111/jog.14316
Sun C., Groom K. M., Oyston C., Chamley L. W., Clark A. R., James J. L. (2020). The placenta in fetal growth restriction: What is going wrong? Placenta 96, 10–18. doi:10.1016/j.placenta.2020.05.003
Szeto H. H., Hinman D. J. (1985). Prenatal development of sleep-wake patterns in sheep. Sleep 8 (4), 347–355. doi:10.1093/sleep/8.4.347
Tagliaferri S., Fanelli A., Esposito G., Esposito F. G., Magenes G., Signorini M. G., et al. (2015). Evaluation of the acceleration and deceleration phase-rectified slope to detect and improve IUGR clinical management. Comput. Math. Methods Med. 2015, e236896. doi:10.1155/2015/236896
Ter Kuile M., Erwich J., Heazell A. E. P. (2021). Stillbirths preceded by reduced fetal movements are more frequently associated with placental insufficiency: A retrospective cohort study. J. Perinat. Med. 50, 668–677. doi:10.1515/jpm-2021-0103
Thompson J. M. D., Wilson J., Bradford B. F., Li M., Cronin R. S., Gordon A., et al. (2021). A better understanding of the association between maternal perception of foetal movements and late stillbirth-findings from an individual participant data meta-analysis. BMC Med. 19 (1), 267. doi:10.1186/s12916-021-02140-z
Unterscheider J., Daly S., Geary M. P., Kennelly M. M., McAuliffe F. M., O'Donoghue K., et al. (2013). Optimizing the definition of intrauterine growth restriction: The multicenter prospective PORTO study. Am. J. Obstet. Gynecol. 208 (4), 290.e1–290.e6. doi:10.1016/j.ajog.2013.02.007
Vasapollo B., Novelli G. P., Farsetti D., Valensise H. (2022). Maternal peripheral vascular resistance at mid gestation in chronic hypertension as a predictor of fetal growth restriction. J. Maternal-Fetal Neonatal Med. 2022, 1–3. doi:10.1080/14767058.2022.2056443
Vinkesteijn A. S., Struijk P. C., Ursem N. T., Hop W. C., Wladimiroff J. W. (2004). Fetal heart rate and umbilical artery flow velocity variability in intrauterine growth restriction: A matched controlled study. Ultrasound Obstet. Gynecol. 23 (5), 461–465. doi:10.1002/uog.1032
Vollgraff Heidweiller-Schreurs C. A., De Boer M. A., Heymans M. W., Schoonmade L. J., Bossuyt P. M. M., Mol B. W. J., et al. (2018). Prognostic accuracy of cerebroplacental ratio and middle cerebral artery Doppler for adverse perinatal outcome: Systematic review and meta-analysis. Ultrasound Obstet. Gynecol. 51 (3), 313–322. doi:10.1002/uog.18809
Vollgraff Heidweiller-Schreurs C. A., van Osch I. R., Heymans M. W., Ganzevoort W., Schoonmade L. J., Bax C. J., et al. (2021). Cerebroplacental ratio in predicting adverse perinatal outcome: A meta-analysis of individual participant data. BJOG Int. J. obstetrics Gynaecol. 128 (2), 226–235. doi:10.1111/1471-0528.16287
Walker A. M., de Preu N. D., Horne R. S., Berger P. J. (1990). Autonomic control of heart rate differs with electrocortical activity and chronic hypoxaemia in fetal lambs. J. Dev. Physiol. 14 (1), 43–48.
Wang K. C., Lim C. H., McMillen I. C., Duffield J. A., Brooks D. A., Morrison J. L. (2013). Alteration of cardiac glucose metabolism in association to low birth weight: Experimental evidence in lambs with left ventricular hypertrophy. Metabolism. 62 (11), 1662–1672. doi:10.1016/j.metabol.2013.06.013
Warrander L. K., Heazell A. E. (2011). Identifying placental dysfunction in women with reduced fetal movements can be used to predict patients at increased risk of pregnancy complications. Med. Hypotheses 76 (1), 17–20. doi:10.1016/j.mehy.2010.08.020
Wassink G., Bennet L., Davidson J. O., Westgate J. A., Gunn A. J. (2013). Pre-existing hypoxia is associated with greater EEG suppression and early onset of evolving seizure activity during brief repeated asphyxia in near-term fetal sheep. PLoS One 8 (8), e73895. doi:10.1371/journal.pone.0073895
Westgate J. A., Wassink G., Bennet L., Gunn A. J. (2005). Spontaneous hypoxia in multiple pregnancies is associated with early fetal decompensation and enhanced T-wave elevation during brief repeated cord occlusion in near-term fetal sheep. Am. J. Obstet. Gynecol. 193 (4), 1526–1533. doi:10.1016/j.ajog.2005.03.024
Whitehead C. L., Cohen N., Visser G. H. A., Farine D. (2020). Are increased fetal movements always reassuring? J. Matern. Fetal. Neonatal Med. 33 (21), 3713–3718. doi:10.1080/14767058.2019.1582027
Wibbens B., Bennet L., Westgate J. A., De Haan H. H., Wassink G., Gunn A. J. (2007). Preexisting hypoxia is associated with a delayed but more sustained rise in T/QRS ratio during prolonged umbilical cord occlusion in near-term fetal sheep. Am. J. Physiol. Regul. Integr. Comp. Physiol. 293 (3), R1287–R1293. doi:10.1152/ajpregu.00373.2007
Wilson D. L., Fung A. M., Skrzypek H., Pell G., Barnes M., Howard M. E., et al. (2022). Maternal sleep behaviours preceding fetal heart rate events on cardiotocography. J. Physiol. 600, 1791–1806. doi:10.1113/JP282528
Wolf H., Gordijn S. J., Onland W., Vliegenthart R. J. S., Ganzevoort J. W. (2020). Computerized fetal heart rate analysis in early preterm fetal growth restriction. Ultrasound Obstet. Gynecol. 56 (1), 51–60. doi:10.1002/uog.21887
Wölter M., Röwer C., Koy C., Rath W., Pecks U., Glocker M. O. (2016). Proteoform profiling of peripheral blood serum proteins from pregnant women provides a molecular IUGR signature. J. Proteomics 149, 44–52. doi:10.1016/j.jprot.2016.04.027
Zur R. L., Kingdom J. C., Parks W. T., Hobson S. R. (2020). The placental basis of fetal growth restriction. Obstet. Gynecol. Clin. North Am. 47 (1), 81–98. doi:10.1016/j.ogc.2019.10.008
Keywords: fetal growth restriction (FGR), stillbirth, fetal hypoxia, biomarkers, fetal heart rate variability (fHRV), preterm brain injury
Citation: King VJ, Bennet L, Stone PR, Clark A, Gunn AJ and Dhillon SK (2022) Fetal growth restriction and stillbirth: Biomarkers for identifying at risk fetuses. Front. Physiol. 13:959750. doi: 10.3389/fphys.2022.959750
Received: 02 June 2022; Accepted: 29 July 2022; Published: 19 August 2022.
Reviewed by:
Copyright © 2022 King, Bennet, Stone, Clark, Gunn and Dhillon. This is an open-access article distributed under the terms of the Creative Commons Attribution License (CC BY). The use, distribution or reproduction in other forums is permitted, provided the original author(s) and the copyright owner(s) are credited and that the original publication in this journal is cited, in accordance with accepted academic practice. No use, distribution or reproduction is permitted which does not comply with these terms.
*Correspondence: Simerdeep K. Dhillon, [email protected]
Disclaimer: All claims expressed in this article are solely those of the authors and do not necessarily represent those of their affiliated organizations, or those of the publisher, the editors and the reviewers. Any product that may be evaluated in this article or claim that may be made by its manufacturer is not guaranteed or endorsed by the publisher.
Thank you for visiting nature.com. You are using a browser version with limited support for CSS. To obtain the best experience, we recommend you use a more up to date browser (or turn off compatibility mode in Internet Explorer). In the meantime, to ensure continued support, we are displaying the site without styles and JavaScript.
- View all journals
- My Account Login
- Explore content
- About the journal
- Publish with us
- Sign up for alerts
- Open access
- Published: 30 March 2024
Paternal DDT exposure induces sex-specific programming of fetal growth, placenta development and offspring’s health phenotypes in a mouse model
- Elaine Chen 1 na1 ,
- Raquel Santana da Cruz 1 na1 ,
- Aallya Nascimento 1 ,
- Meghali Joshi 1 ,
- Duane Gischewski Pereira 1 ,
- Odalys Dominguez 1 ,
- Gabriela Fernandes 1 ,
- Megan Smith 1 ,
- Sara P. C. Paiva 1 , 2 &
- Sonia de Assis ORCID: orcid.org/0000-0001-5053-0614 1
Scientific Reports volume 14 , Article number: 7567 ( 2024 ) Cite this article
Metrics details
- Developmental biology
- Environmental sciences
Mounting evidence suggests that environmentally induced epigenetic inheritance occurs in mammals and that traits in the progeny can be shaped by parental environmental experiences. Epidemiological studies link parental exposure to environmental toxicants, such as the pesticide DDT, to health phenotypes in the progeny, including low birth and increased risk of chronic diseases later in life. Here, we show that the progeny of male mice exposed to DDT in the pre-conception period are born smaller and exhibit sexual dimorphism in metabolic function, with male, but not female, offspring developing severe glucose intolerance compared to controls. These phenotypes in DDT offspring were linked to reduced fetal growth and placenta size as well as placenta-specific reduction of glycogen levels and the nutrient sensor and epigenetic regulator OGT, with more pronounced phenotypes observed in male placentas. However, placenta-specific genetic reduction of OGT only partially replicates the metabolic phenotype observed in offspring of DDT-exposed males. Our findings reveal a role for paternal pre-conception environmental experiences in shaping placenta development and in fetal growth restriction. While many questions remain, our data raise the tantalizing possibility that placenta programming could be a mediator of environmentally induced intergenerational epigenetic inheritance of phenotypes and needs to be further evaluated.
Similar content being viewed by others
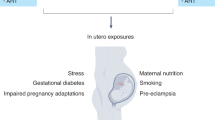
Sex differences in the intergenerational inheritance of metabolic traits
Ionel Sandovici, Denise S. Fernandez-Twinn, … Susan E. Ozanne
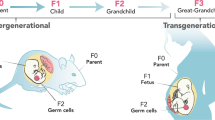
Exploring the evidence for epigenetic regulation of environmental influences on child health across generations
Carrie V. Breton, Remy Landon, … Rebecca Fry
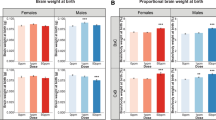
Transcriptomic, proteomic, and metabolomic analyses identify candidate pathways linking maternal cadmium exposure to altered neurodevelopment and behavior
Kathleen M. Hudson, Emily Shiver, … Michael Cowley
Introduction
In eutherian mammals such as human and rodents, the placenta connects the fetus to the mother and provides the nutrient and gas exchanges necessary for optimal fetal development 1 , 2 . It also offers protection from the environment and endocrine support to the pregnancy 1 , 2 , 3 . This extraembryonic organ is derived from the trophectoderm, the structure forming the outer cell layer in pre-implantation embryos at the blastocyst stage 2 . The trophectoderm-derived cell lineage is the first lineage to appear during mammalian development. This cell lineage then gives rise to different placental trophoblast cell types. Trophoblast giant cells, for instance, are crucial for embryo implantation as they allow for infiltration into the maternal uterine epithelium and embryo attachment to the endometrium 2 . Upon implantation, cells in the uterus lining undergo a decidualization process that is critical for normal placentation and embryonic growth and survival 4 .
Although there are organizational differences between the human and the mouse placenta, the role of the major placental structures is quite similar between these two species. They also share comparable gene and protein expression patterns and, consequently, mouse models are considered excellent surrogates to study many aspects of the human placenta 3 . In mice, the placenta contains three main layers. The decidua is derived from the maternal endometrium while the junctional and labyrinth zones are derived from the embryonic trophectoderm 2 . Within the junctional zone, glycogen cells provide energetic and hormonal support for fetal growth. The labyrinth zone is the site of nutrient and gas exchange between mother and fetus 2 , 3 . Defects in both the maternally and embryonically derived layers can cause fetal growth restriction 2 . Abnormal placenta function not only impacts fetal growth but also offspring’s health after birth and throughout the lifespan 3 . A link between placenta dysfunction and maternal health in pregnancy is also well-documented 3 , 5 .
There is accumulating evidence for a placental role in epigenetic inheritance and intergenerational transmission of disease predisposition 3 . Because of the close relationship between mother and fetus, many studies have documented the effects of maternal experiences in pregnancy on placenta development and function 5 , 6 . Yet, despite the growing number of studies showing the importance of pre-conception paternal environmental exposures on offspring’s health, little is known about the impact of paternal experiences on placenta formation, although evidence is emerging 7 , 8 , 9 . Given its embryonic origin, is reasonable to expect that both paternal and maternal factors play a part in placenta development. Surprisingly, seminal studies on imprinting suggest that the process of placenta development is primarily driven by the paternal genome and epigenome while maternal genes are more important in cell and tissue development of the embryo itself 10 , 11 . In support of that, it has been reported that the paternal epigenome has a major impact in placenta formation and that paternally expressed genes are predominant in placenta 12 .
Epidemiological studies link pre-conception paternal exposure to persistence organic pollutants and other environmental chemicals to low birth size in offspring in humans 13 , 14 , 15 , 16 . Low birth weight is often a result of placenta defects, yet there is a lack of studies investigating the link between paternal exposure to environmental toxicants and placenta development.
Here, we developed a mouse model of preconception DDT exposure to investigate whether paternal environmental experiences reprogram placenta development, birth weight and the progeny’s later life health. We found that preconception paternal DDT exposure is linked to a decrease in birth weight and in placenta size as well as placenta-specific suppression of the nutrient sensor and epigenetic regulator OGT. Offspring of DDT-exposed fathers also show a sex-specific increase in metabolic dysfunction. However, placenta-specific genetic reduction of OGT only partially replicates the metabolic phenotype observed in offspring of DDT-exposed males.
Paternal DDT exposure reduces offspring’s birth weight
When mated with unexposed female mice (Fig. 1 a), DDT-exposed males produced offspring that were smaller at birth compared to controls (Fig. 1 b). This is in agreement with human studies showing that parental DDT and other pesticides exposure is linked to low birth weight in offspring 13 . No significant differences in litter size or sex distribution were observed (Fig. 1 c,d). As they aged, DDT offspring underwent a catch-up growth period and beginning at 3 weeks of age, no differences in body weight were observed between the groups (Fig. 1 e,f).
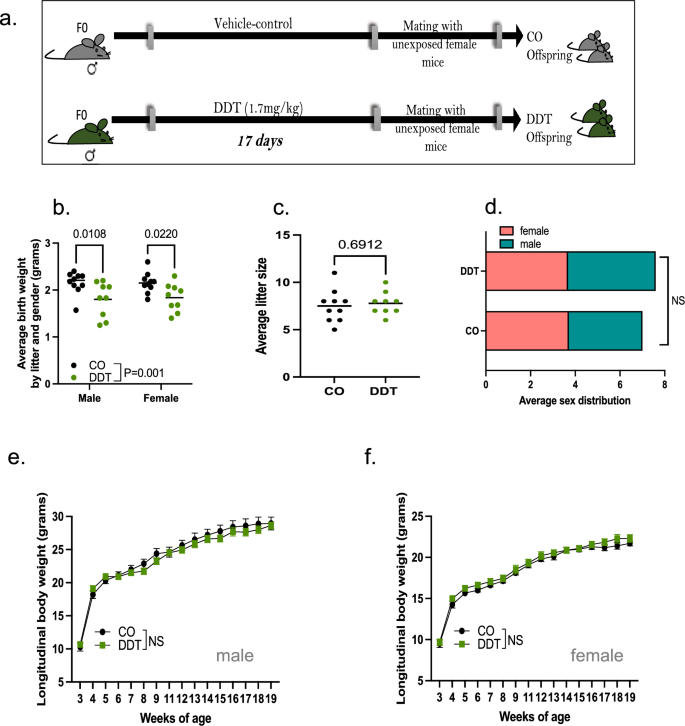
Pre-conception paternal DDT exposure programs offspring’s birth weight. ( a ) Schematic of experimental design: Control (CO) and DDT-exposed male mice were mated to unexposed females to produce offspring. ( b ) Birth weight (grams) of CO and DDT offspring segregated by group and sex. ( c ) Average litter size and ( d ) sex distribution. ( e – f ) Longitudinal body weight (3–19 weeks of age) in male ( e ) and female ( f ) offspring. Data shown as mean (horizontal bars in scatter plots), n = 9–10 litters/group ( b – d ), n = 13–16 males and females/group ( e – f ). Data were analyzed by two-way ANOVA ( b ), t-test ( c ), Fisher's exact test ( d ), or repeated measures ANOVA ( e , f ).
Paternal DDT exposure impairs placenta growth and development
Given the small birth weight in DDT offspring, we next examined the potential impact of pre-conception paternal DDT exposure on fetal growth and placenta development. Pregnant mouse dams mated with either DDT-exposed or control males were euthanized and both placenta and fetal tissues harvested on embryonic day(E) 13.5, after placentation is complete in mice (Fig. 2 a). In agreement with the low birth weight, fetal weights in the DDT group were also reduced with a significant decrease observed in male fetuses only (Fig. 2 b). We also detected a decrease in DDT offspring placenta weights, with more pronounced effects in male placentas (Fig. 2 c). Placental efficiency, defined as grams of fetus produced per gram of placenta, did not differ between the groups for either sex (Fig. 2 d).
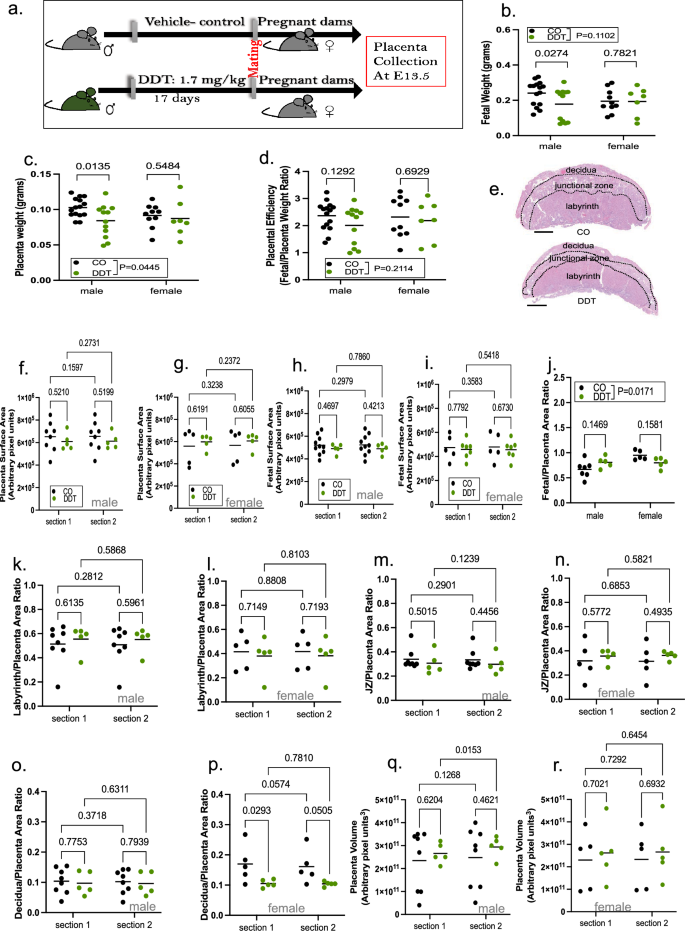
Paternal DDT exposure impairs fetal and placental growth. ( a ) Schematic of experimental design: Control (CO) and DDT-exposed male mice were mated to unexposed females to produce offspring. Pregnant dams were euthanized on E13.5 for placenta collection. ( b ) Fetal weight (grams) by group and sex. ( c ) Placenta weight (grams) by group and sex. ( d ) Placenta efficiency (gram of fetus per gram of placenta) by group and sex. ( e ) Representative picture of mouse H&E-stained placenta showing layers; bar = 0.8 mm. ( f , g ) Placental surface area by group and sex ( f , male; g , female). ( h , i ) Fetal surface area by group and sex ( h , male; i , female). ( j ) Fetus/placenta ratio by group and sex. ( k – p ) Layer-specific placenta surface area normalized to total placenta area and shown by group and sex: ( k , l ) Labyrinth zone ( k , males; l , females), ( m , n ) Junctional zone (JZ) ( m , males; n , females) and ( o , p ) Decidua ( o , males; p , females). ( q , r ) Placental volume by group and sex ( q , males; r , females). Placenta images were analyzed via image J software as shown in Fig. S1 and S3 . Data shown as mean (horizontal bars in scatter plots), n = 19–25/group ( b - d ), n = 5–8/group ( f , k , m , o , q ), n = 5/group ( g , l , n , p , r ), n = 5–10 ( h ), n = 5–7 ( i , j ). Data were analyzed by two-way ANOVA ( b – d , j ) or repeated two-way ANOVA ( f – i , k – r ). section 1 and section 2 labels in f-i and k-r and refer to individual serial sections of each placenta of fetal sample analyzed.
Next, we performed placenta and fetal morphometric analysis using serial histological sections (Fig. S1 a-b, Fig. 2 e). We detected no significant differences in placenta (Fig. 2 f,g) or fetal (Fig. 2 h,i) surface area between the groups. However, we found a significant interaction between sex and group for the fetal to placenta area ratio (Fig. 2 j), with a higher fetus to placenta ratio for DDT male placentas but a smaller ratio for DDT female placentas compared to controls.
It has been reported that defects in different placenta zones are associated with fetal growth restriction 2 . We next quantified the surface area for specific placenta layers (Fig. S2 a-c). When normalizing the area for each placental layer to the total placenta surface area (Fig. 2 k–p), we found no significant differences in the labyrinth (Fig. 2 k,l) or junctional (Fig. 2 m,n) zones between the groups. However, we observed a significant decrease in the decidua zone in female, but not male, DDT placentas compared to controls (Fig. 2 o,p). We also calculated placenta volume from measurements of placenta width (Fig. S2 d-e), height (Fig. S2 f-g) and thickness (Fig. S2 h-i), but did not find significant differences between the groups (Fig. 2 q,r).
Paternal DDT exposure reduces placenta glycogen content and expression and activity of O-GlcNAc transferase (OGT)
Glucose is the major nutrient source for energy generation in the placenta. The glycogen cells within the placenta provides energetic support to the fetus 2 . We quantified placental glycogen content via PAS-amylase assay and normalized it by total placenta area (Fig. S4 ). We found a significant reduction in placental glycogen levels, independent of sex, in the DDT group compared to controls (Fig. 3 a–c).
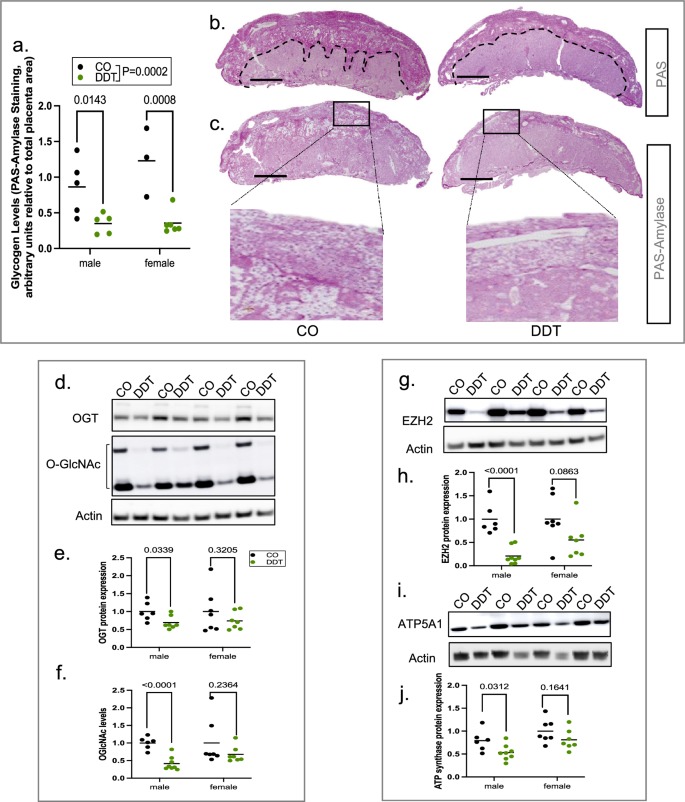
Paternal DDT exposure reduces placenta glycogen levels and expression and activity of O-GlcNAc transferase (OGT). Pregnant dams were euthanized on E13.5 for placenta collection. ( a ) Placenta glycogen level quantification (PAS-amylase staining, normalized by placenta area) by group and sex. ( b , c ) Representative pictures of PAS ( b ) or PAS-amylase ( c ) stained mouse placentas. Top area delineated by dashed lines in b depicts regions with strong PAS staining. PAS-amylase stain inset in c shows glycogen storing cells (glycogen stores appear white in section); bar = 1 mm; Images were analyzed via image J software as shown in Fig. S4 . ( d – f ) Placenta OGT expression and O-GlcNAc levels: ( d – f ) Representative Western-Blots (males) showing OGT expression and O-GlcNAcylation levels with beta-actin as loading control; ( e – f ) Quantification of placenta OGT and O-GlcNAc levels by group and sex ( e , f ). ( g , h ) Placenta EZH2 expression levels: ( g ) Representative Western-Blots (males) showing EZH2 expression with beta-actin as loading control; ( h ) Quantification of placenta EZH2 expression by group and sex. ( i , j ) Placenta ATP synthase (ATP5A1) expression levels: ( i ) Representative Western-Blots (males) showing ATP5A1expression with beta-actin as loading control; ( j ) Quantification of placenta ATP5A1by group and sex. Data shown as mean (horizontal bars in scatter plots), n = 8–11/group (glycogen levels); n = 13–15/group (western-blots). Data were analyzed by two-way ANOVA ( a ) or t-test ( e , f , h , j ). Full-length Western-Blots membranes (male and female placentas) are shown in Fig. S5 - 6 .
Reasoning that reduced glycogen levels could disrupt energy sensing activity in placenta, we next examined the levels and activity of OGT (O-Linked N-Acetylglucosamine [GlcNAc] transferase). This enzyme is a nutrient sensor and its activity is dependent on glucose flux through the hexosamine biosynthetic pathway 17 , 18 . OGT plays an important role in placenta development and function 19 . Indeed, we found that both OGT protein levels and OGT-catalyzed protein O- GlcNAcylation were reduced in placentas of DDT offspring (Fig. 3 d–f).
OGT is an X-linked gene. Placenta displays sexual dimorphism in X-linked genes becasue X chromosome inactivation is malleable in female placentas 20 . Indeed, we found that paternal DDT associated reduction in placental OGT expression and O-GlcNAcylation was sex-specific and more pronounced in male placentas (Fig. 3 e,f). Importantly, sexual dimorphism in the expression of OGT has also been reported in human placentas 6 .
As a O-GlcNAc transferase, OGT plays a key role in many biological processes via posttranslational modification and stabilization of both cytosolic and nuclear proteins 18 . For instance, OGT controls chromatin remodeling by mediating histone methylation via stabilization of the histone methyltransferase EZH2 21 . Consistent with lower levels of OGT and O-GlcNAcylation, levels of EZH2 protein were significantly reduced (Fig. 3 g,h) in placentas of DDT offspring, particularly in male placentas.
Another documented target of OGT is ATP synthase subunit alpha (ATP5A1) 22 , a protein that is part of a complex that controls energy levels in the form of ATP to fulfill the bioenergetic function of the placenta. Although not as pronounced as EZH2, we also found a sex-specific reduction of ATP synthase protein levels, with a more marked decreased in DDT male offspring placentas compared to controls (Fig. 3 i,j).
Paternal DDT exposure programs metabolic dysfunction in offspring
Small birth weight has been associated with chronic diseases such diabetes later in life 23 . Given this relationship, we next examined whether paternal DDT exposure increased metabolic dysfunction in the progeny ages 8–10 week of age. Indeed, DDT offspring showed poor metabolic function in adulthood, with a sex-specific effect (Fig. 4 a–i). Male DDT offspring displayed severe glucose intolerance compared to controls (Fig. 4 b,d). In contrast, female DDT offspring showed a small but significant better glucose handling than control females (Fig. 4 c,e).
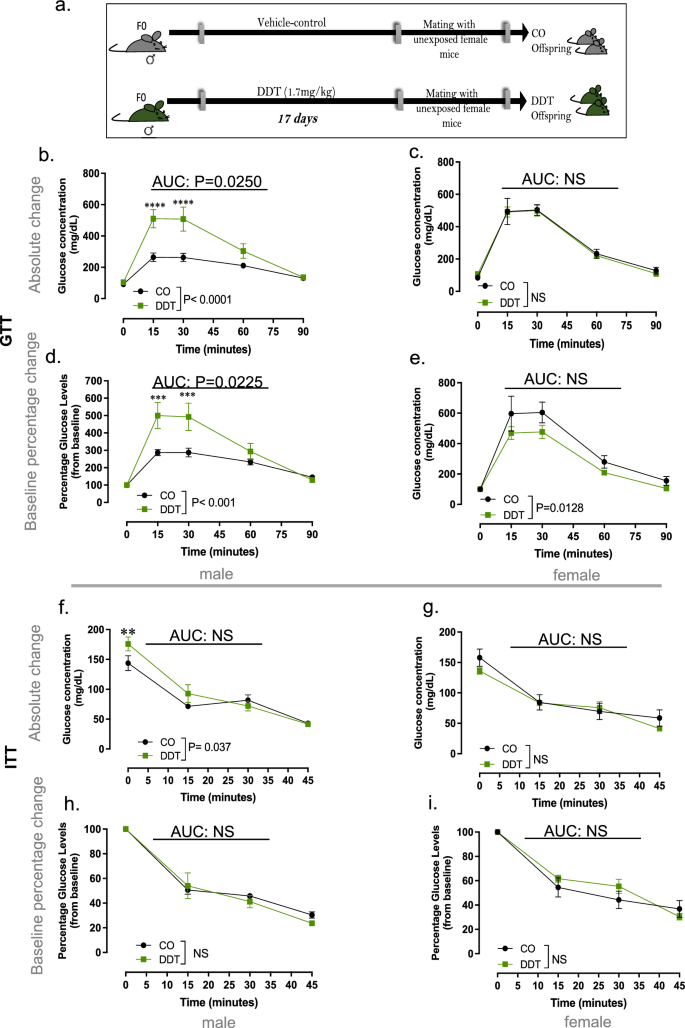
Paternal DDT exposure programs metabolic dysfunction in offspring. ( a ) Schematic of experimental design: Control (CO) and DDT-exposed males were mated to unexposed females to produce offspring. Metabolic function in offspring of CO and DDT-exposed males at 8–10 weeks of age: ( b – e ) Glucose tolerance test (GTT) shown as absolute glucose values ( b , c ) or percentage change from baseline ( d , e ) in male ( b , d ) and female ( c , e ) offspring; ( f – i ) Insulin Tolerance Test (ITT) shown as absolute glucose values ( f - g ) or percentage change from baseline ( h , i ) in male ( f , h ) and female ( g , i ) offspring. Data shown as mean ± SEM; n = 9 males and females/group ( b – e ), n = 8–9 males and females /group ( f – i ). GTT or ITT curves were analyzed by repeated measures ANOVA. AUC data was analyzed by t-test.
Although male DDT offspring had higher baseline glucose levels after a 4-h fast, no differences in insulin sensitivity were observed between the groups for either males or females. (Fig. 4 f–i).
Placenta-specific genetic deletion of OGT partially replicates the effects of paternal DDT on offspring metabolic function in a sex specific manner.
To examine whether suppression of OGT activity in placenta was functionally linked to programming of metabolic dysfunction in DDT progeny, we used a mouse Cre -Lox system for placenta-specific genetic deletion of OGT (Figs. 5 a–h and 6 a–h).
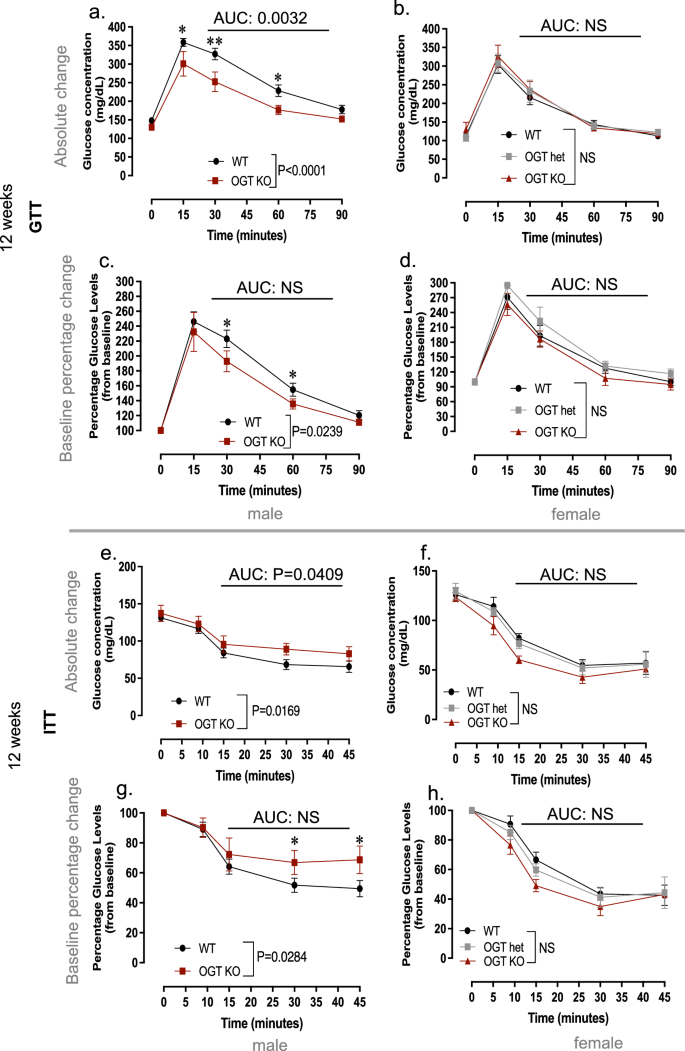
Placenta-specific genetic deletion of OGT does not fully replicate the effects of paternal DDT on offspring metabolic function at 12 weeks of age. ( a – h ) Metabolic function in WT and OGTKO mice at 12 weeks of age: ( a – d ) Glucose tolerance test (GTT) shown as absolute glucose values ( a , b ) or percentage change from baseline ( c , d ) in male ( a , c ) and female ( b , d ) offspring; ( e – h ) Insulin Tolerance Test (ITT) shown as absolute glucose values ( e – f ) or percentage change from baseline ( g , h ) in male ( e , g ) and female ( f , h ) offspring. Data shown as mean ± SEM; ( a – d ) n = 21 (WT males and females), n = 9 (OGTKO males and females), n = 5 (OGThet); ( e – h ) n = 19 (WT males and females), n = 9 (OGTKO males and females), n = 6 (OGThet females); ITT and GTT data were analyzed by repeated measures ANOVA ( a – h ). AUC data was analyzed by t-test ( a , c , e , g ) or one-way ANOVA ( b , d , f , h ).
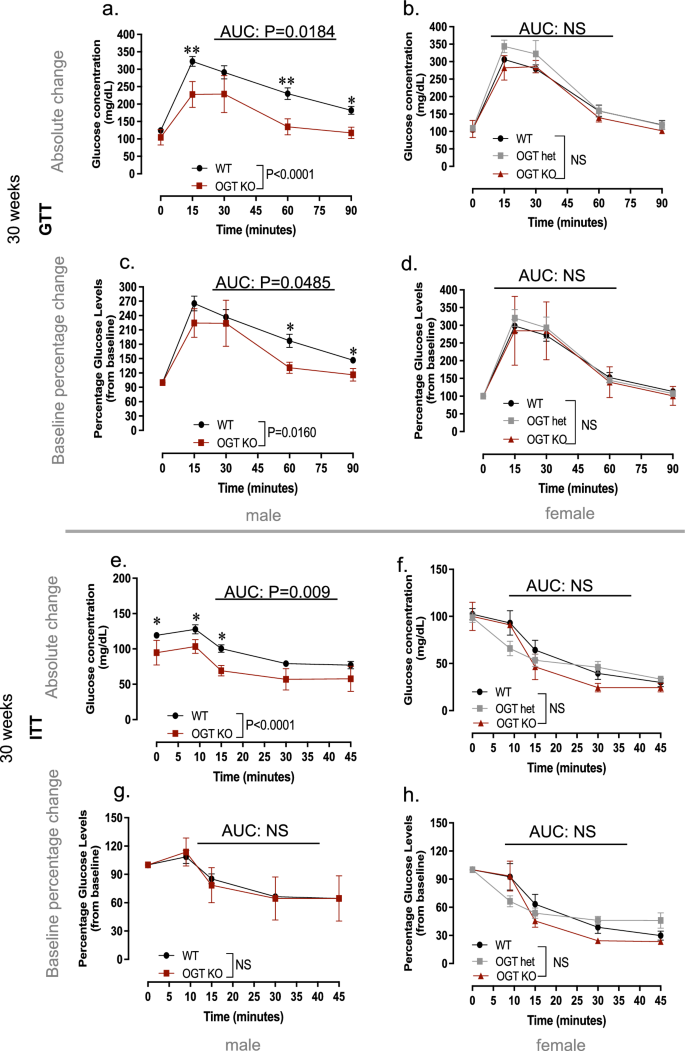
Placenta-specific genetic deletion of OGT does not replicate the effects of paternal DDT on offspring metabolic function at 30 weeks of age. ( a – h ) Metabolic function in WT and OGTKO mice at 30 weeks of age: ( a – d ) Glucose tolerance test (GTT) shown as absolute glucose values ( a , b ) or percentage change from baseline ( c , d ) in male ( a , c ) and female ( b , d ) offspring; ( e – h ) Insulin Tolerance Test (ITT) shown as absolute glucose values ( e , f ) or percentage change from baseline ( g , h ) in male ( e , g ) and female ( f , h ) offspring. Data shown as mean ± SEM; ( a - h ) n = 16 (WT males and females), n = 5 (OGTKO males and females), n = 8 (OGThet females); ITT and GTT data was analyzed by repeated measures ANOVA ( a - h ). AUC data was analyzed by t-test ( a , c , e , g ) or one-way ANOVA ( b , d , f , h ). ITT and GTT data were analyzed by repeated measures ANOVA ( a – h ). AUC data was analyzed by t-test ( a , c , e , g ) or one-way ANOVA ( b , d , f , h ).
Though male offspring with placenta-specific OGT deletion (OGTKO/Y) genotype showed metabolic dysfunction, their phenotype only partially replicated that of DDT male offspring (Fig. 5 a,c,e,g). While placenta OGTKO/Y male offspring had higher absolute glucose levels after a 4-h fast and an increase in insulin resistant in early adulthood (Fig. 5 e,g), they showed better glucose handling than WT control male littermates (Fig. 5 a,c). Female offspring with placenta-specific OGT reduction (OGTKO or OGThet) showed no signs of metabolic dysfunction compared to WT littermates at 12 weeks of age (Fig. 5 b,d,f,h).
Because metabolic dysfunction tends to worsen with age 24 , we repeated metabolic assessments at 30 weeks of age (Fig. 6 a–h). Placenta OGTKO/Y males continued to show better glucose tolerance compared to WT males (Fig. 6 a,c). However, no differences in insulin sensitivity were observed between males with different placenta genotypes, despite the lower baseline glucose levels in OGTKO/Y males at this age (Fig. 6 e,g). Metabolic function in placenta OGT KO or OGThet female offspring did not differ from WT littermates at 30 week of age (Fig. 6 b,d,f,h).
Placenta-specific genetic deletion of OGT impacts offspring body-weight gain with age in a sex specific manner
To better understand what metabolic phenotypes observed in offspring with placenta-specific OGT reduction, we evaluated their body weight compared to WT mice from weaning to 30 weeks of age (Fig. 7 a–d). While placenta OGTKO/Y and WT males displayed similar body weight gain until about 12 weeks of age, OGTKO/Y males did not gain as much body weight after this time point compared to WT controls, weighing significantly less by 24 weeks of age (Fig. 7 a,c). Not surprisingly, there were no differences in body weight in placenta OGTKO or OGThet female offspring compared to WT controls (Fig. 7 b,d).
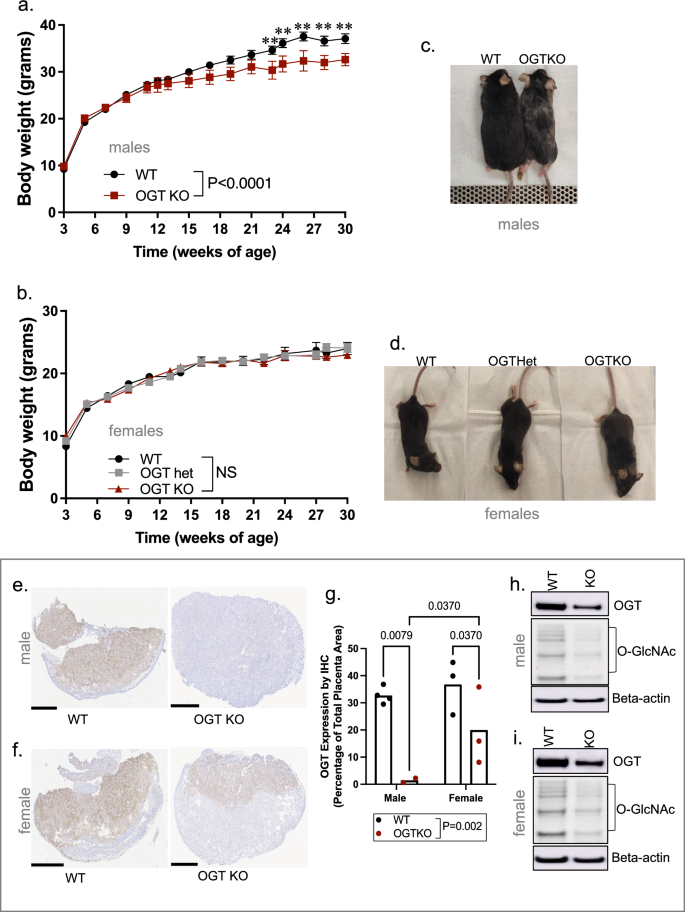
Placenta-specific genetic deletion of OGT impacts offspring’s bodyweight in a sex specific manner. ( a , b ) Longitudinal body weight (3–30 weeks of age) in ( a ) WT or OGTKO males and in ( b ) WT, OGThet or OGTKO females. Representative pictures showing ( c ) WT or OGTKO males and ( d ) WT males OGThet or OGTKO females at 30 weeks of age. ( e , f ) Representative pictures showing OGT protein immunostaining in placentas of ( e ) males or ( f ) females with a WT or OGTKO genotype; bar = 1 mm; ( g ) Quantification of placenta OGT protein immunostaining by group and sex. ( h , i ) Representative Western-Blots showing OGT protein expression and O-GlcNAcylation levels in placentas of ( h ) males or ( i ) females with a WT or OGTKO genotype; beta-actin, loading control. Data shown as mean ± SEM ( a , b ) or mean ( g ); Body weight data were analyzed by repeated measures ANOVA; n = 16 (WT males and females), n = 5 (OGTKO males and females), n = 8 (OGThet females). OGT immunostaining quantification was analyzed by two-way ANOVA; n = 5 (WT placentas), n = 7 (OGTKO placentas). Full-length Western-Blot membranes are shown Fig. S7.
Because OGT is an X-linked gene, we wondered whether placenta Cre recombinase mediated OGT deletion is more efficient in males than in females in this model. We found that this is indeed the case. In OGTKO/Y male placentas, OGT protein expression and activity is almost completely lost (Fig. 7 e,g,h). While placenta OGTKO females showed a decreased in OGT expression and activity (O-GlcNAcylation levels) compared to the WT group (Fig. 7 f,g,i), they still retained higher expression levels of OGT protein compared to OGTKO/Y male placentas.
Using a mouse model, we found that preconception paternal DDT exposure is linked to a decrease in the progeny’s birth weight and reduced placental size. We also observed a reduction in placental glycogen levels and the nutrient sensor and epigenetic regulator OGT, with more pronounced phenotypes observed in male placentas. The progeny of DDT-exposed males also exhibited sexual dimorphism in metabolic function, with DDT male offspring developing severe glucose intolerance while DDT female offspring show slightly better glucose handling compared to controls. However, placenta-specific genetic reduction of OGT only partially replicates the metabolic phenotype observed in offspring of DDT-exposed males.
Our findings are consistent with epidemiological studies linking parental exposure to persistence organic pollutants and other environmental chemicals to low birth size in offspring in humans 13 , 14 , 15 , 16 , 25 . In our model, the reduced birth weight in offspring of DDT-exposed males was associated with impaired fetal growth and placenta weight.
There is accumulating evidence for placenta as a mediator of environmentally-induced intergenerational transmission of disease 3 . Given the tight connection between the fetus and the mother during gestation, it is well documented that maternal experiences in pregnancy impact placenta development and function 5 , 6 . In our paradigm, paternal exposure to the environmental toxicant DDT also impaired placenta development, its glycogen storage and nutrient sensing signaling.
In humans and mice, the inner cell mass and the trophectoderm— the layer containing cells that give rise the placenta and other extraembryonic tissues— are the first definitive embryonic cell lineages to appear 3 . The integrity and function of the trophectoderm is critical for the blastocyst-stage embryo implantation and pregnancy success. Defective placentation can result in fetal growth restriction and other pregnancy complications 26 , 27 . Although details need to be elucidated, our data suggest that pre-conception paternal environmental exposures can contribute to disruption of this process and lead to defective placentation.
Abnormal placenta function and adverse events in fetal development have been associated with disease later in life. In this study, we found that paternal exposure to DDT also results in metabolic dysfunction and poor glucose handling in the male, but not female, progeny. Interestingly, both human and animal studies link maternal exposure to DDT to metabolic dysfunction in the next generations 28 , 29 , 30 . These findings, along with previously published reports, suggest that paternal exposures may be as important as maternal ones regarding determination of the progeny’s phenotypes. This striking overlap between the impact of maternal or paternal exposure on offspring phenotypes has been noted by others 31 , and our current findings raise the possibility that the placenta may be the common mediator of intergenerational epigenetic inheritance of phenotypes.
OGT is encoded by an X linked-gene and is a key regulator of several biological processes through its posttranslational modification of both nuclear and cytosolic proteins. Unlike other somatic tissues, the inactivation of the X chromosome in the placenta is plastic, allowing for its reactivation in response to stress 20 , 32 . It has been proposed that this is the reason why female fetuses can be more resilient to environmental stressors 33 . Our findings are in line with this concept as paternal DDT exposure has greater impact on placenta of male fetuses and suppresses placenta OGT signaling in a sex-specific manner. Importantly, this sexual dimorphism in placenta OGT expression has also been reported for maternal exposures 6 , further strengthening the notion that placenta abnormalities could mediate both paternal and maternal effects on the progeny.
In our study, placenta OGT reduction did not fully replicate the metabolic dysfunction observed in DDT offspring. It is possible that the divergent metabolic phenotypes between DDT offspring and placenta OGT KO offspring is due to timing. While in the placenta OGTKO mouse model used here, Cre-induced genetic knockdown occurs early in development 34 , it’s possible that OGT suppression in placenta of DDT offspring is a later event in the placentation process, though this still needs to be investigated. In males, the X chromosome is maternally inherited. This further supports the notion that placental reduction in OGT expression and activity observed in DDT male progeny, which also show abnormal glucose homeostasis, is a secondary event and not directly inherited from the paternal genome. Yet, we cannot completely rule out that other factors inherited from the paternal sperm play a role.
OGT and its biochemical mark, O-GlcNAcylation, play a role in placentation through its regulation of syncytiotrophoblast formation. In BeWo trophoblast cells, OGT depletion via siRNA leads to spontaneous differentiation 35 . Although it does not completely phenocopy the poor glucose handling in DDT male offspring, it is possible that OGT genetic reduction in the early trophectoderm causes a more serious disruption in placentation than observed in the paternal DDT paradigm. This likely leads to suboptimal fetal development and more severe health phenotypes such as impaired body weight gain and neurodevelopmental disorders 6 later in life. While not described in this report, our early findings suggest that placenta OGT genetic deletion results in in many other health alterations in offspring as they age.
Why OGT genetic reduction results in sex specific effects on metabolic homeostasis is not entirely clear. However, the fact longitudinal body weight of OGTKO and OGThet females does not differ from the control littermates unlike OGTKO/Y males offers a clue. We also showed that OGTKO females retain some degree of OGT expression in placenta, likely due to incomplete recombination, which could be enough to provide protection. It is possible that the sexual dimorphism observed in body weight in older OGTKO offspring could be due to accelerated aging in male mice, though confirmation studies are needed. Interestingly, in mouse models of aging, impaired body weight gain is caused by loss of bone and muscle mass. In these models, sex-specific difference has been reported because estrogens can protect female mice from sarcopenia 36 . It has also been reported that placenta EZH2, an OGT target, is responsible for the female fetus resilience to maternal stress 37 . However, whether this epigenetic modifier plays a role in the observed sex-specific phenotypes still need to be investigated.
Employing the same placenta-specific OGTKO mouse model used here, a recent study 38 found no differences in glucose homeostasis in males 6–8 weeks of age or fed a high-fat diet, but reported an increase in insulin sensitivity in OGThet placenta females after a 12-week course of high fat diet. This was associated with increased hepatic insulin/Akt axis signaling. The study also reported a decrease in pancreatic islet size for mice with placenta-specific OGT knockout compared to the controls but this was compensated by an increase in the total number of islets. While both of our studies found better glucose handling in mice with placenta-specific OGT reduction, this phenotype was detected exclusively in males in the present study. Differences between our studies likely stem from the timing of metabolic testing and different dietary conditions. In the present study, mice were kept on chow diet throughout the study while in the Moore et al. study, mice were switched to a high-fat diet at 12 weeks of age. We also backcrossed the original FBV Cyp19-Cre mice into the c57bl6 background, which could further explain the phenotypic differences between the two studies.
A recurring finding in our study is the sex-specific outcomes with more pronounced phenotypes in male DDT offspring. This is supported by other literature reports showing that the impact of parental exposures on offspring health is often sexually-dimorphic. In humans, in utero tobacco smoke exposure is linked to greater severity of disease in females 39 . In rodents, female offspring from obese mothers show better hepatic functioning that protect them from adverse health effects observed in males 40 . In contrast, female offspring of fathers exposed to morphine have worse memory deficits compared to male offspring 41 . This parentally-induced sexual dimorphism on offspring’s health extends to placenta phenotypes and seems dependent on the environmental stressor. Animal models show that maternal exposure to benzene or stress leads to sex-specific transcriptional changes in placentas 42 , 43 while paternal obesity leads to placenta hypoxia in female placentas 9 .
Sex differences in progeny’s phenotypes in response to parental experiences can be attributed to sexual dimorphism associated with sex chromosomes. Placenta X chromosome inactivation (XCI) is imprinted with preferential inactivation of the paternal X chromosome. However, selective inactivation of the paternal X chromosome in female placenta has been reported and can lead to gene dosage differences between the sexes. The OGT gene, for instance, has been show to escape XCI and show higher expression in female placenta in both humans and mice 43 , 44 , 45 . Additional mechanisms that contribute to sex-specific differences in offspring phenotypes include sex hormones (which can impact the both gene expression and gut microbiota) and difference in mitochondrial function 33 , 45 , 46 .
While we showed a link between paternal DDT exposure and programming of placenta development, the environmentally-induced paternal factors involved in placenta development need to be fully evaluated. In our model, mating of DDT-exposed males with unexposed females was performed overnight and offspring and placenta traits likely stem from changes in the sperm epigenome as reported before. The impact of environmental factors on the sperm epigenome, particularly sperm RNAs, has been well documented by us and others 47 , 48 , 49 , 50 , 51 . However, whether and how the sperm epigenome plays a role in placenta development remains to be investigated. DDT has been reported to be a mutagen at very high doses 52 and though the low DDT dose used in this study is likely non-genotoxic, we cannot completely rule out genetic abnormalities in paternal sperm.
Our study also has other limitations. The Cyp19-Cre line used to genetically suppress OGT expression in placenta can be inefficient. Two recent publications report that Cyp19-Cre activity in placenta is quite variable, with only about a third of maternally inherited Cyp19-Cre leading to recombination levels of 70% or greater 53 , 54 . In addition, we cannot completely rule out a confounding effect of the mixed genetic background in OGTKO offspring, which could have impacted placental OGT expression as well as the progeny’s metabolic phenotypes. Thus, results obtained using the OGTKO model require conservative interpretation.
Despite these limitations and remaining questions, our study show that preconception paternal DDT induced alteration in offspring is linked to placenta defects. Our findings, along with recent reports 7 , 9 , raise the tantalizing possibility that placenta programming is a mediator of environmentally induced intergenerational inheritance of phenotypes and needs to be further evaluated.
Materials and Methods
Ddt exposure and breeding.
The C57BL/6NTac strain of mice (Taconic Biosciences) was used in this experiment. Adult male mice (8 weeks of age) were either treated daily with a DDT solution (1.7 mg/kg) or vehicle-control solution (peanut oil) via oral gavage for 17 days. Duration of exposure was chosen in order to encompass the entire period of post-testicular sperm transit in the epididymis (which takes about 15 days in mammals), the last stage of sperm maturation 55 . The DDT solution used mimics the formulation of DDT before its ban in the U.S.: 77.2% p,p’-DDT and 22.8% o,p’-DDT as described before 29 .
At the end of the exposure period, DDT or vehicle-control exposed male mice were mated overnight to unexposed females to generate the offspring. Pregnant dams were allowed to deliver their offspring or euthanized on embryonic day (E)13.5 for placenta collection as described below. All mice utilized in this study were kept on a standard chow diet including the exposures and breeding periods, for the extent of pregnancy (21 days) and after birth. To avoid litter-effect, pups were cross-fostered one day after birth. All pups were weaned on postnatal day (PND) 21. The offspring of control-vehicle or DDT-exposed fathers were used to study metabolic function as described in the following sections.
The number of offspring/litters stemming from different fathers included in each experimental endpoint is shown in Table S1 .
All animal procedures were approved by the Georgetown University Animal Care and Use Committee, and the experiments were performed following the National Institutes of Health guidelines for the proper and humane use of animals in biomedical research. Animals were randomized to each exposure group and treatments were performed blindly. The study is reported in accordance with ARRIVE guidelines.
Placenta harvest
Non-exposed female mice were mated with DDT or vehicle-control exposed males overnight as described above. Mating was confirmed by detection of a vaginal plug. This was considered the first day of pregnancy or embryonic day (E) 0.5. Pregnant dams were housed in groups of two with free access to food and water and euthanized either on E13.5, after placentation is complete in mice. Placentas and fetuses were weighed upon dissection and either snap frozen or fixed in 10% neutral-buffered formalin. DNA from fetal tail tips were used for gender determination via commercial genotyping (Transnetyx, Inc.) of the Y chromosome specific gene, SRY.
Placenta morphometric, cellular and molecular assessments
Morphometric assessment.
Placenta surface area, width, height, and thickness and volume were evaluated using two placenta sections per sample in the Image J Fiji image processing software. Morphometric ends points were total placental surface area and surface area of each placental layer (labyrinth, junctional zone and maternal decidua). The detailed methodology is shown in Fig. S1 a. Briefly, an image outline of each individual mid-sagittal serial placenta section (PAS staining) was traced using the Image J software tracing function. The number of pixels in the selected area was then calculated by the software. These procedures were then repeated to determine the area of each specific placenta layer.
We also estimated the dimensions (width, height, and thickness) of each mid-sagittal placenta section image, using Image J software tracing function and resulting number of pixels as illustrated in Fig. S3 . Placenta volume was calculated from measurements of width, height, and thickness using a formula adapted from Azpurua et al. 56 .
To assess placental efficiency, defined as the gram of fetus per gram of placenta, the weight of each individual fetus was divided by its placenta weight.
Glycogen assessment
Placenta glycogen content was measured via periodic acid–Schiff (PAS) staining, with or without α-amylase digestion, using a commercial PAS staining system (Sigma-Aldrich, catalog #395), according to manufacturer’s instructions. To quantify placenta glycogen levels, two serial PAS-stained placenta sections (with and without alpha-amylase treatment) were analyzed using the ImageJ Fiji image processing software. Using the H PAS function within ImageJ, color filters were separated, and then the intensity of PAS staining (pixels) was quantified for both section images. Glycogen levels were measured using the difference in color intensity between images without and with amylase and normalized by total placenta area (Fig. S4 ).
Molecular assessments
Protein levels of OGT, EZH2, ATP5A1 and patterns of protein O-GlcNAcylation were assessed via Western-Blot (as described below) in placenta (See Table S2 for list antibodies and dilutions).
The number of samples/litters stemming from different fathers included in placenta endpoint is shown in Table S1 .
Fetal morphometric assessment
Fetal surface area was assessed using two embryo sections per sample in the Image J Fiji image processing software. The detailed methodology is shown in Fig. S1 b. Briefly, an outline of each individual mid-sagittal fetal section image (H&E staining) was traced using the Image J software tracing function. The number of pixels in the selected area was then calculated by the software.
Assessment of longitudinal body weight and metabolic parameters in offspring
Offspring of vehicle-control or DDT exposed males were weighed one day after birth and weekly starting at weaning (3 weeks of age) and monitored until 20 weeks of age.
Glucose tolerance test (GTT) and insulin tolerance test (ITT) were performed in 8 to10 week-old mice. For the GTT, mice were fasted for 6 h, injected i.p. with glucose (2.5 g/kg) and glucose measurements taken at 0, 15, 30, 60, and 90 min using a Accu-check meter. For the ITT, mice were fasted for 4 h, injected i.p. insulin (0.75 U/kg), glucose measurements at 0, 15, 30, 45, 60 and 90 min using an Accu-check meter. GTT and ITT curves were prepared using either the absolute glucose levels at each time point or the percentage glucose change from baseline (time zero) at each time point.
The number of offspring/litters stemming from different fathers included in metabolic endpoints is shown in Table S1 .
Placenta-specific genetic reduction of OGT
A mouse Cre-Lox system under the control of the Cyp19-Cre promoter was used in this experiment. For the placenta-specific reduction of OGT, Ogt tm1Gwh /(X OGTflox /X WT ); CYP19-Cre (placental-Cre recombinase homozygote, P. Cre +/+ )] females were mated to hemizygous [Ogt tm1Gwh /(X OGTflox /Y)] males , resulting in offspring of the following genotypes as described before 57 : functional WT females (X WT /X WT /Cre + or X WT /X WT /Cre − or X OGT /X WT /Cre), OGThet female (X OGT /X WT /Cre + ), OGTKO female (X OGT /X OGT /Cre + ), functional WT/Y males (X WT /Y/Cre + or X WT /Y/Cre − ) or male OGTKO/Y (X OGT /Y/Cre + ). In this mouse model, CYP19-Cre is expressed throughout the junctional and labyrinth trophoblast in placentas 34 . CYP19-Cre mice ( FVB strain) used in this study was a gift from Dr. Gustavo Leone from the Medical College of Wisconsin. These mice were back crossed to the C57BL/6NTac strain for at least 5 generations before being used in this study. The Ogt tm1Gwh mice were obtained from Jackson laboratories (Strain #:004860) and have a B6.129 (C57BL/6; 129P3/J) mixed background.
Resulting offspring were used for body weight and metabolic studies as described in the next section. Another cohort of pregnant dams were euthanized before giving birth and used for placenta collection to confirm OGT genetic reduction via western blot or immunohistochemistry (described in the next sections). Offspring’s genotyping (OGT and SRY) was carried out commercially (via tail clipping) by Transnetyx, Inc.
Longitudinal assessment of body weight and metabolic parameters in placenta specific WT or OGTKO offspring
Offspring were weighted weekly starting at weaning. Glucose tolerance test (GTT) and insulin tolerance test (ITT) were performed at 11–12 weeks of age and then again at 30 weeks of age. For the GTT, mice were fasted for 6 h, injected i.p. with glucose (2.5 g/kg) and glucose measurements taken at 0, 15, 30, 60, and 90 min using a Accu-check meter. For the ITT, mice were fasted for 4 h, injected i.p. insulin (0.75 U/kg), glucose measurements at 0, 9, 15, 30 and 45 min using an Accu-check meter. GTT and ITT curves were prepared using either the absolute glucose levels at each time point or the percentage glucose change from baseline (time zero) at each time point.
Western-blots
Total protein was extracted from placental tissues using RIPA buffer with Halt™ Protease Inhibitor Cocktail (Thermo Fisher). Protein extracts were resolved on a 4–12% denaturing polyacrylamide gel (SDS-PAGE). Proteins were transferred using the iBlot® 7-Minute Blotting System (Invitrogen, USA) and blocked with 5% non-fat dry milk for 1 h at room temperature. Membranes were incubated with the specific primary antibodies (for antibody specifications and dilutions see Table S2 ) at 4 °C overnight. After several washes, the membranes were incubated with horseradish peroxidase (HRP)-conjugated secondary antibody at room temperature for 1 h. Membranes were developed using the Chemiluminescent HRP antibody detection reagent (Denville Scientific Inc., USA), and exposed to blot imaging systems (Amersham™ Imager 600, GE Healthcare Life Sciences). Optical density of the bands was quantified using Quantity-one software (BIO-RAD, USA). To control for equal protein loading, expression of the proteins of interest was normalized to the β-actin signal.
Immunohistochemistry
Five microns sections from formalin fixed paraffin-embedded placenta tissues were de-paraffinized with xylenes and rehydrated through a graded alcohol series. Heat induced epitope retrieval (HIER) was performed by immersing the tissue sections in Target Retrieval Solution, Low pH (DAKO) in the PT Link (DAKO). Immunohistochemical staining was performed using a horseradish peroxidase labeled polymer (Agilent , K4003) according to manufacturer’s instructions. Briefly, slides were either treated with 3% hydrogen peroxide and 10% normal goat serum for 10 min each, and exposed to primary antibody for OGT (1:200, Cell Signaling, D1D8Q) for 1 h at room temperature. Slides were exposed to the appropriate HRP labeled polymer for 30 min and DAB chromagen (Dako) for 5 min. Slides were counterstained with Hematoxylin (Fisher, Harris Modified Hematoxylin), blued in 1% ammonium hydroxide, dehydrated, and mounted with Acrymount. Consecutive sections with the primary antibody omitted were used as negative controls.
Statistical analysis
Statistical analyses were performed using GraphPad Prism (GraphPad Software, San Diego, CA, USA). Normal probability plots were used to ascertain normality, which were confirmed by Anderson–Darling, D’Agostino-Pearson, Kolmogorov–Smirnov or Shapiro–Wilk tests. Where data failed the normality test, log transformation was performed prior to statistical analysis or a non-parametric test used. Repeated measures two-way ANOVA was used to analyze longitudinal body weight curves, ITT, GTT, placenta and fetal morphometric endpoints (group, time or serial section). Two-way ANOVA was used to analyze birth weight, placenta weight, glycogens levels (group, sex or layer). Sex distribution was analyzed by Fisher’s exact test. All other end-points were analyzed using unpaired t-test (for two groups) or one-way ANOVA (for three groups). Differences were considered statistically significant at P < 0.05. Unless indicated, n corresponds to the number of animals used in each experiment. The number of samples/litters stemming from different fathers included in each endpoint is shown in Table S1 .
Data availability
The datasets used and/or analyzed during the current study available from the corresponding author on reasonable request.
Hemberger, M., Hanna, C. W. & Dean, W. Mechanisms of early placental development in mouse and humans. Nat. Rev. Genet. 21 , 27–43. https://doi.org/10.1038/s41576-019-0169-4 (2020).
Article CAS PubMed Google Scholar
Woods, L., Perez-Garcia, V. & Hemberger, M. Regulation of placental development and its impact on fetal growth-new insights from mouse models. Front Endocrinol (Lausanne) 9 , 570. https://doi.org/10.3389/fendo.2018.00570 (2018).
Article PubMed Google Scholar
Nugent, B. M. & Bale, T. L. The omniscient placenta: Metabolic and epigenetic regulation of fetal programming. Front. Neuroendocrinol. 39 , 28–37. https://doi.org/10.1016/j.yfrne.2015.09.001 (2015).
Article CAS PubMed PubMed Central Google Scholar
Ramathal, C. Y., Bagchi, I. C., Taylor, R. N. & Bagchi, M. K. Endometrial decidualization: Of mice and men. Semin. Reprod. Med. 28 , 17–26. https://doi.org/10.1055/s-0029-1242989 (2010).
Rosenfeld, C. S. Sex-specific placental responses in fetal development. Endocrinology 156 , 3422–3434. https://doi.org/10.1210/en.2015-1227 (2015).
Howerton, C. L., Morgan, C. P., Fischer, D. B. & Bale, T. L. O-GlcNAc transferase (OGT) as a placental biomarker of maternal stress and reprogramming of CNS gene transcription in development. Proc. Natl. Acad. Sci. USA 110 , 5169–5174. https://doi.org/10.1073/pnas.1300065110 (2013).
Article ADS PubMed PubMed Central Google Scholar
Watkins, A. J. et al. Paternal low protein diet programs preimplantation embryo gene expression, fetal growth and skeletal development in mice. Biochimica et Biophysica Acta https://doi.org/10.1016/j.bbadis.2017.02.009 (2017).
Binder, N. K. et al. Paternal obesity in a rodent model affects placental gene expression in a sex-specific manner. Reproduction 149 , 435–444. https://doi.org/10.1530/REP-14-0676 (2015).
Jazwiec, P. A. et al. Paternal obesity induces placental hypoxia and sex-specific impairments in placental vascularization and offspring metabolismdagger. Biol. Reprod. 107 , 574–589. https://doi.org/10.1093/biolre/ioac066 (2022).
Article PubMed PubMed Central Google Scholar
Barton, S. C., Surani, M. A. & Norris, M. L. Role of paternal and maternal genomes in mouse development. Nature 311 , 374–376. https://doi.org/10.1038/311374a0 (1984).
Article ADS CAS PubMed Google Scholar
Surani, M. A., Barton, S. C. & Norris, M. L. Development of reconstituted mouse eggs suggests imprinting of the genome during gametogenesis. Nature 308 , 548–550. https://doi.org/10.1038/308548a0 (1984).
Wang, X., Miller, D. C., Harman, R., Antczak, D. F. & Clark, A. G. Paternally expressed genes predominate in the placenta. Proc. Natl. Acad. Sci. USA 110 , 10705–10710. https://doi.org/10.1073/pnas.1308998110 (2013).
Robledo, C. A. et al. Preconception maternal and paternal exposure to persistent organic pollutants and birth size: The LIFE study. Environ. Health Perspect. 123 , 88–94. https://doi.org/10.1289/ehp.1308016 (2015).
Zhang, H. et al. Potential occupational exposure of parents to endocrine disrupting chemicals, adverse birth outcomes, and the modification effects of multi-vitamins supplement and infant sex. Ecotoxicol. Environ. Saf. 233 , 113314. https://doi.org/10.1016/j.ecoenv.2022.113314 (2022).
Zang, L. et al. Association of phthalate exposure with low birth weight in couples conceiving naturally or via assisted reproductive technology in a prospective birth cohort. Sci. Total Environ. 855 , 158852. https://doi.org/10.1016/j.scitotenv.2022.158852 (2023).
Zhang, Y. et al. Association of Parental Preconception Exposure to Phthalates and Phthalate Substitutes With Preterm Birth. JAMA Netw Open 3 , e202159. https://doi.org/10.1001/jamanetworkopen.2020.2159 (2020).
Bond, M. R. & Hanover, J. A. A little sugar goes a long way: The cell biology of O-GlcNAc. J Cell Biol 208 , 869–880. https://doi.org/10.1083/jcb.201501101 (2015).
Yang, X. & Qian, K. Protein O-GlcNAcylation: Emerging mechanisms and functions. Nat. Rev. Mol. Cell Biol. 18 , 452–465. https://doi.org/10.1038/nrm.2017.22 (2017).
Lima, V. V. et al. O-GlcNAc modification during pregnancy: Focus on placental environment. Front. Physiol. 9 , 1263. https://doi.org/10.3389/fphys.2018.01263 (2018).
Dubois, A. et al. Spontaneous reactivation of clusters of X-linked genes is associated with the plasticity of X-inactivation in mouse trophoblast stem cells. Stem Cells 32 , 377–390. https://doi.org/10.1002/stem.1557 (2014).
Chu, C. S. et al. O-GlcNAcylation regulates EZH2 protein stability and function. Proc. Natl. Acad. Sci. USA 111 , 1355–1360. https://doi.org/10.1073/pnas.1323226111 (2014).
Article ADS CAS PubMed PubMed Central Google Scholar
Cha, M. Y. et al. Mitochondrial ATP synthase activity is impaired by suppressed O-GlcNAcylation in Alzheimer’s disease. Hum. Mol. Genet. 24 , 6492–6504. https://doi.org/10.1093/hmg/ddv358 (2015).
Boehmer, B. H., Limesand, S. W. & Rozance, P. J. The impact of IUGR on pancreatic islet development and beta-cell function. J. Endocrinol. 235 , R63–R76. https://doi.org/10.1530/JOE-17-0076 (2017).
Gouveia, E. R. et al. Predictors of metabolic syndrome in adults and older adults from Amazonas, Brazil. Int. J. Environ. Res. Public Health https://doi.org/10.3390/ijerph18031303 (2021).
Kezios, K. L. et al. Dichlorodiphenyltrichloroethane (DDT), DDT metabolites and pregnancy outcomes. Reprod. Toxicol. 35 , 156–164. https://doi.org/10.1016/j.reprotox.2012.10.013 (2013).
Roland, C. S. et al. Morphological changes of placental syncytium and their implications for the pathogenesis of preeclampsia. Cell Mol Life Sci 73 , 365–376. https://doi.org/10.1007/s00018-015-2069-x (2016).
Ruebner, M. et al. Reduced syncytin-1 expression levels in placental syndromes correlates with epigenetic hypermethylation of the ERVW-1 promoter region. PLoS ONE 8 , e56145. https://doi.org/10.1371/journal.pone.0056145 (2013).
La Merrill, M. A., Krigbaum, N. Y., Cirillo, P. M. & Cohn, B. A. Association between maternal exposure to the pesticide dichlorodiphenyltrichloroethane (DDT) and risk of obesity in middle age. Int. J. Obes. (Lond.) 44 , 1723–1732. https://doi.org/10.1038/s41366-020-0586-7 (2020).
La Merrill, M. et al. Perinatal exposure of mice to the pesticide DDT impairs energy expenditure and metabolism in adult female offspring. PLoS ONE 9 , e107332. https://doi.org/10.1371/journal.pone.0103337 (2014).
Article CAS Google Scholar
Skinner, M. K. et al. Ancestral dichlorodiphenyltrichloroethane (DDT) exposure promotes epigenetic transgenerational inheritance of obesity. BMC Med. 11 , 228. https://doi.org/10.1186/1741-7015-11-228 (2013).
Rando, O. J. & Simmons, R. A. I’m eating for two: Parental dietary effects on offspring metabolism. Cell 161 , 93–105. https://doi.org/10.1016/j.cell.2015.02.021 (2015).
Migeon, B. R., Axelman, J. & Jeppesen, P. Differential X reactivation in human placental cells: Implications for reversal of X inactivation. Am. J. Hum. Genet. 77 , 355–364. https://doi.org/10.1086/432815 (2005).
Bronson, S. L. & Bale, T. L. The placenta as a mediator of stress effects on neurodevelopmental reprogramming. Neuropsychopharmacology 41 , 207–218. https://doi.org/10.1038/npp.2015.231 (2016).
Wenzel, P. L. & Leone, G. Expression of Cre recombinase in early diploid trophoblast cells of the mouse placenta. Genesis 45 , 129–134. https://doi.org/10.1002/dvg.20276 (2007).
Panetta, P. Characterization of O-GlcNAc signalling in human placenta. Ph.D. thesis, University of Warwick (2021). http://webcat.warwick.ac.uk/record=b3598087
Xie, W. Q. et al. Mouse models of sarcopenia: Classification and evaluation. J. Cachexia Sarcopenia Muscle 12 , 538–554. https://doi.org/10.1002/jcsm.12709 (2021).
Nugent, B. M., O’Donnell, C. M., Epperson, C. N. & Bale, T. L. Placental H3K27me3 establishes female resilience to prenatal insults. Nat. Commun. 9 , 2555. https://doi.org/10.1038/s41467-018-04992-1 (2018).
Moore, M., Avula, N., Jo, S., Beetch, M. & Alejandro, E. U. Disruption of O-linked N-acetylglucosamine signaling in placenta induces insulin sensitivity in female offspring. Int. J. Mol. Sci. 22 , 6918. https://doi.org/10.3390/ijms22136918 (2021).
Reddy, K. D. & Oliver, B. G. G. Sex-specific effects of in utero and adult tobacco smoke exposure. Am. J. Physiol. Lung Cell Mol. Physiol. 320 , L63–L72. https://doi.org/10.1152/ajplung.00273.2020 (2021).
Savva, C. et al. Molecular programming modulates hepatic lipid metabolism and adult metabolic risk in the offspring of obese mothers in a sex-specific manner. Commun. Biol. 5 , 1057. https://doi.org/10.1038/s42003-022-04022-3 (2022).
Ellis, A. S. et al. Paternal morphine self-administration produces object recognition memory deficits in female, but not male offspring. Psychopharmacology (Berl) 237 , 1209–1221. https://doi.org/10.1007/s00213-019-05450-6 (2020).
Maxwell, A. et al. Intrinsic sexual dimorphism in the placenta determines the differential response to benzene exposure. iScience 26 , 106287. https://doi.org/10.1016/j.isci.2023.106287 (2023).
Bale, T. L. The placenta and neurodevelopment: Sex differences in prenatal vulnerability. Dialogues Clin. Neurosci. 18 , 459–464 (2016).
Hemberger, M. Epigenetic landscape required for placental development. Cell Mol. Life Sci. 64 , 2422–2436. https://doi.org/10.1007/s00018-007-7113-z (2007).
Sandovici, I., Fernandez-Twinn, D. S., Hufnagel, A., Constancia, M. & Ozanne, S. E. Sex differences in the intergenerational inheritance of metabolic traits. Nat. Metab. 4 , 507–523. https://doi.org/10.1038/s42255-022-00570-4 (2022).
Christians, J. K. The Placenta’s role in sexually dimorphic fetal growth strategies. Reprod. Sci. 29 , 1895–1907. https://doi.org/10.1007/s43032-021-00780-3 (2022).
Sharma, U. et al. Biogenesis and function of tRNA fragments during sperm maturation and fertilization in mammals. Science 351 , 391–396. https://doi.org/10.1126/science.aad6780 (2016).
Chen, Q., Yan, W. & Duan, E. Epigenetic inheritance of acquired traits through sperm RNAs and sperm RNA modifications. Nat. Rev. Genet. 17 , 733–743. https://doi.org/10.1038/nrg.2016.106 (2016).
Wang, Y. et al. Sperm microRNAs confer depression susceptibility to offspring. Sci. Adv. 7 , eabd7605. https://doi.org/10.1126/sciadv.abd7605 (2021).
Grandjean, V. et al. RNA-mediated paternal heredity of diet-induced obesity and metabolic disorders. Sci. Rep. 5 , 18193. https://doi.org/10.1038/srep18193 (2015).
da Cruz, R. S. et al. Paternal malnutrition programs breast cancer risk and tumor metabolism in offspring. Breast Cancer Res. 20 , 99. https://doi.org/10.1186/s13058-018-1034-7 (2018).
Clark, J. M. Mutagenicity of DDT in mice, Drosophila melanogaster and Neurospora crassa . Aust. J. Biol. Sci. 27 , 427–440. https://doi.org/10.1071/bi9740427 (1974).
Anamthathmakula, P. et al. Variable Cre recombination efficiency in placentas of Cyp19-Cre ROSA(mT/mG) transgenic mice. Cells 12 , 2096. https://doi.org/10.3390/cells12162096 (2023).
Sandovici, I. et al. The imprinted Igf2-Igf2r axis is critical for matching placental microvasculature expansion to fetal growth. Dev. Cell 57 , 63–79 e68. https://doi.org/10.1016/j.devcel.2021.12.005 (2022).
James, E. R. et al. The role of the epididymis and the contribution of epididymosomes to mammalian reproduction. Int. J. Mol. Sci. 21 , 5377. https://doi.org/10.3390/ijms21155377 (2020).
Azpurua, H. et al. Determination of placental weight using two-dimensional sonography and volumetric mathematic modeling. Am. J. Perinatol. 27 , 151–155. https://doi.org/10.1055/s-0029-1234034 (2010).
Howerton, C. L. & Bale, T. L. Targeted placental deletion of OGT recapitulates the prenatal stress phenotype including hypothalamic mitochondrial dysfunction. Proc. Natl. Acad. Sci. USA 111 , 9639–9644. https://doi.org/10.1073/pnas.1401203111 (2014).
Download references
Acknowledgements
We thank the following Lombardi Cancer Center Shared Resources (SR) for their assistance: Animal Model SR, Histopathology & Tissue SR, Microscopy and Imaging SR. We also thank Ms. M. Idalia Cruz for her technical assistance with animal experiments.
This study was supported by the National Institutes of Health (ES031611 to Sonia de Assis and P30-CA51008; Lombardi Comprehensive Cancer Center Support Grant to Louis Weiner), and by the Georgetown-Howard Universities Georgetown-Howard Universities Center for Clinical and Translational Science (GHUCCTS) (National Center for Advancing Translational Sciences of the National Institutes of Health, UL1TR000101 , Pilot Award to Sonia de Assis). Gabriela Fernandes was supported in part by the CAPES Foundation-Brazil (PDSE- 88881.624565/2021-01).
Author information
These authors contributed equally: Elaine Chen and Raquel Santana da Cruz.
Authors and Affiliations
Department of Oncology, Lombardi Comprehensive Cancer Center, Georgetown University, Washington, DC, 20057, USA
Elaine Chen, Raquel Santana da Cruz, Aallya Nascimento, Meghali Joshi, Duane Gischewski Pereira, Odalys Dominguez, Gabriela Fernandes, Megan Smith, Sara P. C. Paiva & Sonia de Assis
Department of Obstetrics and Gynecology, Federal University of Minas Gerais (UFMG), Belo Horizonte, MG, Brazil
Sara P. C. Paiva
You can also search for this author in PubMed Google Scholar
Contributions
E.C. and R.S.D.C. supervised the animal work and tissue collection and performed molecular analysis; A.N., M. J., and D. G. P. completed a portion of the animal work and placenta analysis. O.D., G. F. and M.S. assisted with animal studies, processed tissues and/or helped perform molecular analysis; S. P.C. P. contributed with data analysis and wrote the manuscript; S.D.A. conceived the study, oversaw the research and wrote the manuscript.
Corresponding author
Correspondence to Sonia de Assis .
Ethics declarations
Competing interests.
The authors declare no competing interests.
Additional information
Publisher's note.
Springer Nature remains neutral with regard to jurisdictional claims in published maps and institutional affiliations.
Supplementary Information
Supplementary tables., supplementary figures., rights and permissions.
Open Access This article is licensed under a Creative Commons Attribution 4.0 International License, which permits use, sharing, adaptation, distribution and reproduction in any medium or format, as long as you give appropriate credit to the original author(s) and the source, provide a link to the Creative Commons licence, and indicate if changes were made. The images or other third party material in this article are included in the article's Creative Commons licence, unless indicated otherwise in a credit line to the material. If material is not included in the article's Creative Commons licence and your intended use is not permitted by statutory regulation or exceeds the permitted use, you will need to obtain permission directly from the copyright holder. To view a copy of this licence, visit http://creativecommons.org/licenses/by/4.0/ .
Reprints and permissions
About this article
Cite this article.
Chen, E., da Cruz, R.S., Nascimento, A. et al. Paternal DDT exposure induces sex-specific programming of fetal growth, placenta development and offspring’s health phenotypes in a mouse model. Sci Rep 14 , 7567 (2024). https://doi.org/10.1038/s41598-024-58176-7
Download citation
Received : 14 December 2023
Accepted : 26 March 2024
Published : 30 March 2024
DOI : https://doi.org/10.1038/s41598-024-58176-7
Share this article
Anyone you share the following link with will be able to read this content:
Sorry, a shareable link is not currently available for this article.
Provided by the Springer Nature SharedIt content-sharing initiative
By submitting a comment you agree to abide by our Terms and Community Guidelines . If you find something abusive or that does not comply with our terms or guidelines please flag it as inappropriate.
Quick links
- Explore articles by subject
- Guide to authors
- Editorial policies
Sign up for the Nature Briefing newsletter — what matters in science, free to your inbox daily.

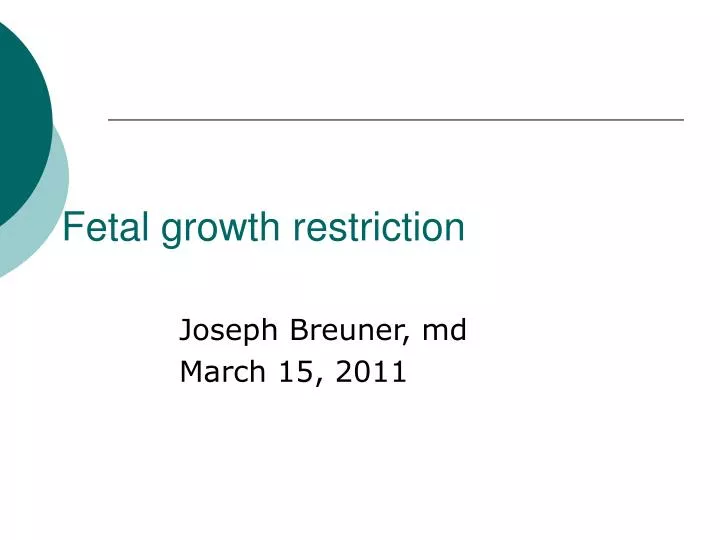
Fetal growth restriction
Dec 22, 2011
610 likes | 1.34k Views
Fetal growth restriction. Joseph Breuner, md March 15, 2011. Teaching points. 16 wk rule Small vs in trouble Safer out or in: <34 wks use normal UA flow by DV to continue >34 wks deliver if Maternal htn Growth arrest x 3-4 wks Bpp low (<6/8) DV UA reversed or absent. Teaching points.
Share Presentation
- differentiate
- systolic diastolic ratio
- abdominal size liver volume
- uteroplacental insufficiency

Presentation Transcript
Fetal growth restriction Joseph Breuner, md March 15, 2011
Teaching points • 16 wk rule • Small vs in trouble • Safer out or in: • <34 wks use normal UA flow by DV to continue • >34 wks deliver if • Maternal htn • Growth arrest x 3-4 wks • Bpp low (<6/8) • DV UA reversed or absent
outline Risk factors Diagnosis Evaluation management
Risk factors Fetal Placental maternal
fetal • Genetic-SGA parent, sibling • Chromosomal abnormality • Congenital anomalies • Multiple gestation • infection
Placental risk factors • Ischemic placental disease=preeclampsia, FGR, abruption or combination • Small placenta- • Confined placental mosaicism
Maternal factors 1 • Uteroplacental insufficiency: htn, renal dz, diabetes, CVD, SLE, antiphospholipid syndrome, preeclampsia • Diminished caloric intake • Hypoxemia-pulm dz, cyanotic heart dz, severe anemia, high altitude
Maternal factors 2 • Hematologic d/o-sickle • Substance use-smoking, alcohol, stimulants • Toxins-meds warfarin, anticonvulsants, chemo, mtx • Caffeine • radiation
Maternal factors 3 • ART • Uterine malformations • Extremes of reproductive age • Short interpregnancy interval
16 week rule 1st 16 weeks: all cell division, uniform 2nd 16 weeks (16-32) combo cell division and growth, much more variability 32-40 primarily cell growth
definition <10%ile (50-70% will be constitutionally small) Some malnourished fetuses will be >10th%ile <5%ile Severe<3%ile
GA • Needs early US • Accuracy of Ultrasound decreases with advancing GA: • 1st TM: ± 3-5 days • 14-20 weeks: ± 7-10 days • 20-26 weeks: 2-3 weeks • Beyond 36 weeks: 3-4.5 weeks
Fundal height • Define by > 2cm discrepancy after 20 wks • Best when • Same clinician • Unmarked side of tape
Symmetric/asymmetric Symmetric-all organs decreasd proportionally due to hyperplasia(division) impairment Asymmetric-relatively greater decrease in abdominal size(liver volume/sq fat) than head circumference. Redistribution of blood flow to head
Symmetric-3 causes • Constitutionally small • Aneuploidy/congenital anomalies • Early infection
asymmetric • Always substrate deficiency
For fetuses <10th%ile 70% 20% 10%
For fetuses <5th%ile 40% 40% 20%
For fetuses <3th%ile 20% 53% 27%
ultrasound Crucial tool to Confirm/exclude dx Differentiate symmetric/asymmetric Verify fetal tolerance/well being
biometry Purpose of biometry is to detect FGR
Who gets an ultrasound? • >2cm FH discrepancy once • 2 cm FH discrepancy more than once • Or following RF’s
US Screening for IUGR • Previous IUGR fetus • Maternal medical condition • CHTN, autoimmune disorders, asthma, APS • Multiples • Uterine anomaly • Placental abnormality • Marginal/velamentous cord • Ongoing bleeding • Chronic abruption, previa • Abnormal serum screening analytes
AC most sensitive 3616 preg >25 wks gestation Single us within two wks delivery Predicted <10th%ile for GA with Sens 61% Spec 95% Ppv 86% Npv 83%
AC • More predictive of FGR than • HC • BPD • Combination
AC • More sensitive in asymmetric than symmetric GR (73%vs59%) • GA-more sensitive later in gestation • Sn/PPV at 29-31 wks 41/51%, at term 88/71% • More sensitive when interval between measurements >2wks. FP rates for interexam intervals 1,2,4 wks 31,17,3%
EFW Best algorithm uses ac,bpd,fl EFW within 10% of actual bw 75% of time Sn/sp/ppv/npv 90/85/80/90 Sens increases with worsening GR Log10 BW = 1.335 - 0.0034(AC)(FL) + 0.0316(BPD) +0.0457(AC) + 0.1623(FL)
Growth velocity • Small vs in trouble • Falling %iles shd raise alarm
ratios • HC/AC • FL/AC • TCD/AC
Diagnosis summary • Of four entities described-AC, EFW, growth velocity, and ratios • Dx depends on EFW • Log10 BW = 1.335 - 0.0034(AC)(FL) + 0.0316(BPD) +0.0457(AC) + 0.1623(FL)
Diagnosis summary • 10%ile-at risk • 5th%ile act • 3rd%ile
Evaluation and management • H+P-etoh,tobacco, maternal vasc dz • Anatomic survey/fetal echo • Karyotype for <32 wks, <3rd %ile, polyhydramnios or structural anomalies • Ab testing for cmv/rubella/vzv only if maternal symptoms suggestive or US shows echo/calcification of liver, brain, or hydrops
follow • Fetal wt for growth velocity q 2-4 wks • BPP 1-2x/wk • Amniotic fluid volume weekly (part of bpp) • doppler
doppler • Cochrane review • Doppler vs no doppler in FGR pregnancies • Reduced perinatal deaths by 29% (OR 0.71, CI 0.5-1.01)
Umbilical artery • Increasing systolic/diastolic ratio, f/b • Absent or reversed end-diastolic flow
Middle cerebral artery • Reported as umbilical/intracranial ratio, or • Peak velocity of middle cerebral artery
Umbilical vein or ductus venosus • Ductus venosus flow reverses • Umbilical vein develops pulsatile flow
Sample us report from inpt • Indication: IUGR with estimated weight on the 3rd percentile on 3/11/2011. Admitted for treatment. Comparison: 12/3/2010 through 3/11/2011. Expected menstrual age of 32 weeks 5 days with EDD 5/3/2011. The fetus is vertex with an anterior normal appearing placenta of normal thickness. The amniotic fluid is decreasing. AFI is 6 to 7 today done 3 times. The AFI was 10 on 3/11/2011. Fetal Doppler: Performed because of IUGR The umbilical arterial systolic diastolic ratio of 2.5 is normal. The umbilical/intracranial Doppler ratio of 0.5 is normal. The ductus venosus waveform was technically difficult to obtain because of fetal position, but limited adequate samples appear normal.
Sample us report • Based on EDD 5/13/2011, the expected menstrual age is 31 weeks. Twin live intrauterine fetuses identified. The presenting twin is in breech position and the nonpresenting twin is in cephalic position. Confluent posterior placenta is identified. Separating membrane is visualized. • The amount of amniotic fluid appears normal for twin A with the amniotic fluid index of 11. The amount of amniotic fluid appears normal for twin B with the amniotic fluid index of 12. There is no evidence for hydrops fetalis for either twin. • OB DOPPLER performed, per request, because of monochorionicity. Twin A: Umbilical artery Doppler shows normal systolic to diastolic ratio of 2.5. Umbilical/intracranial Doppler ratio of 0.4 is normal. Middle cerebral artery peak systolic velocity 55 cm/sec is 1.3 multiples of the median and normal. Twin B: Umbilical artery Doppler shows normal systolic to diastolic ratio of 2.9. Umbilical/intracranial Doppler ratio of 0.4 is normal. Middle cerebral artery peak systolic velocity 42 cm/sec is 1.0 multiples of the median and normal.
steroids • Two large studies conflict • Reasonable to administer • Likely the very impaired fetus can’t respond
Delivery timing • Growth Restriction Intervention Trial (GRIT) • 580 women 24-34 wks • Randomly assigned to immediate or delayed delivery groups if ob uncertain when to intervene • 90%FGR • 40% absent or reversed end diast UA flow
GRIT • Immediate delivery: when ob uncertain • Delayed delivery: when ob no longer uncertain (average delay 4.9d) • Deaths prior to hospital d/c same 29/27 • Immediate fewer stillbirths (2 v 9) but more neonatal/infant deaths (27 v 18)
Recommended management • Remote from term <34 wks • Follow doppler. Prolong pregnancy if UA flow normal. Deliver if absent/reversed • Some experts await abnormal venous flow in very preterm-investigational
- More by User
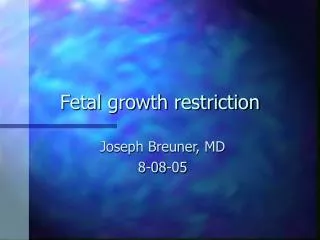
Fetal growth restriction Joseph Breuner, MD 8-08-05 Objectives Define risk factors Define screening Define diagnosis Define management Take-home points Risk factors: if positive, obtain ultrasound for growth 16-24 wks if negative, use fundal height to screen Take-home points
1.66k views • 44 slides
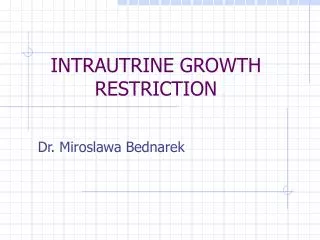
INTRAUTRINE GROWTH RESTRICTION
INTRAUTRINE GROWTH RESTRICTION. Dr. Miroslawa Bednarek. Diagnosis of symptom. Fetal growth failing (arising from maternal, placental, or fetal origins)
919 views • 28 slides
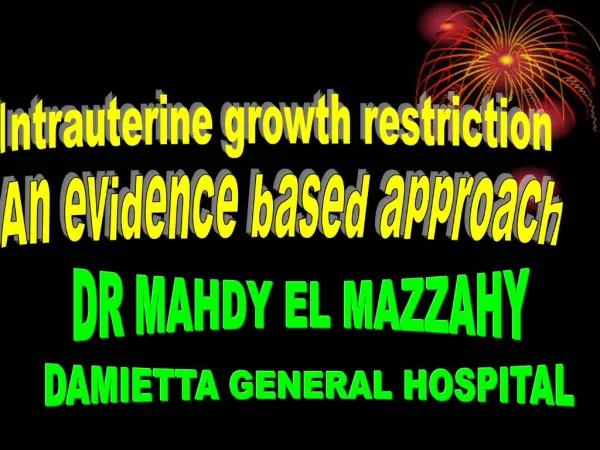
Intrauterine growth restriction
Introduction and background . IUGR remains a challenging problem for both the obstetrician and pediatrician. The ability to diagnose the disorder and understand its pathophysiology still outpaces the ability to prevent its complications.. . Introduction and background. . Fetal growth restriction is
1.14k views • 62 slides
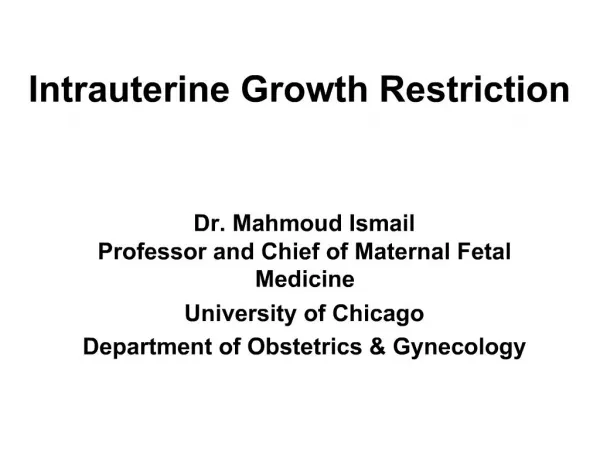
Intrauterine Growth Restriction
I.U.G.R.. DefinitionTypes of IUGREtiologyFeto placentalMaternalDiagnosisFetal MeasurementsOligohydramniosMaternal Doppler Velocimetry. I.U.G.R. ManagementAntepartumFetal GrowthFetal Well BeingTherapyTiming of DeliveryNeonatal outcomeImmediate MorbidityLong Term Morbidity. I.U.G.R.. D
898 views • 62 slides
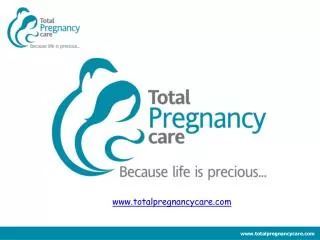
Intra-uterine Growth Restriction
742 views • 44 slides
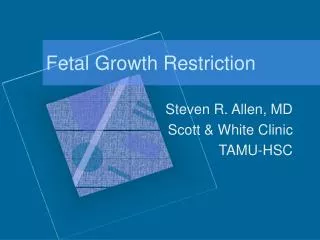
Fetal Growth Restriction
Fetal Growth Restriction. Steven R. Allen, MD Scott & White Clinic TAMU-HSC. Educational Objectives. Review epidemiology and significance of fetal growth restriction (FGR) Know etiologies (= risk factors) Discuss screening strategies for FGR
765 views • 42 slides
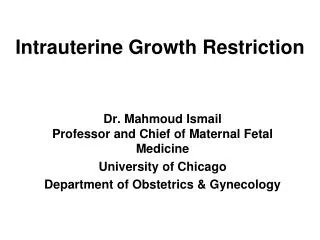
Intrauterine Growth Restriction. Dr. Mahmoud Ismail Professor and Chief of Maternal Fetal Medicine University of Chicago Department of Obstetrics & Gynecology. I.U.G.R. Definition Types of IUGR Etiology Feto placental Maternal Diagnosis Fetal Measurements Oligohydramnios
1.55k views • 62 slides
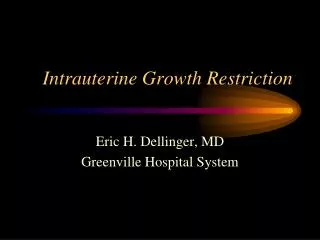
Intrauterine Growth Restriction. Eric H. Dellinger, MD Greenville Hospital System. IUGR: Introduction. IUGR 2nd leading contributor to PNM rate PNM rate increased 6-10 fold PNM rate 8/1000 background: 120/1000 for all IUGR 60-80/1000 when anomalies excluded. IUGR: Introduction.
1.1k views • 31 slides
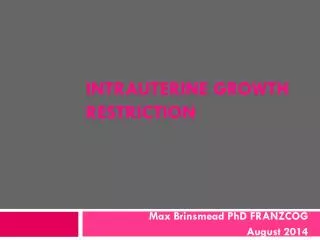
INTRAUTERINE GROWTH RESTRICTION
INTRAUTERINE GROWTH RESTRICTION. Max Brinsmead PhD FRANZCOG August 2014. The fetus is unique because. He or she cannot signal his or health by way of any history And we can only examine through his or her mother We can only... Document size and growth Evaluate his or her movements
1.17k views • 19 slides
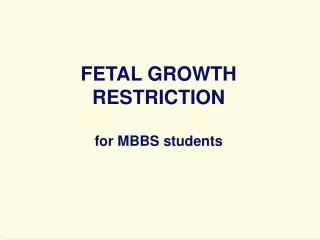
FETAL GROWTH RESTRICTION for MBBS students
FETAL GROWTH RESTRICTION for MBBS students. Definition. Fetuses that have failed to achieve their growth potential because of inadequate oxygen and nutritional supply. FGR is divided into two groups. Type 1: Fetus is symmetrically small Type2:Fetal growth is asymmetrical.
792 views • 36 slides
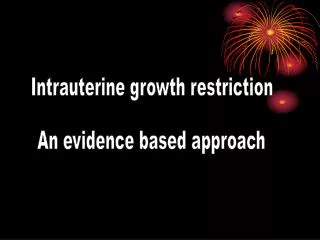
Intrauterine growth restriction. An evidence based approach. Introduction and background. IUGR remains a challenging problem for both the obstetrician and pediatrician.
1.43k views • 62 slides
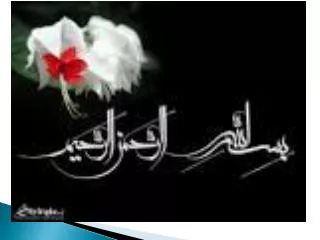
Small for gestational age fetal growth restriction low birth weight
Small for gestational age fetal growth restriction low birth weight. 29/11/92 Mojgan Barati. Definition. For the purposes of this guideline, SGA birth is defined as an estimated fetal weight (EFW) or abdominal circumference (AC) less than the 10th centile and
482 views • 17 slides
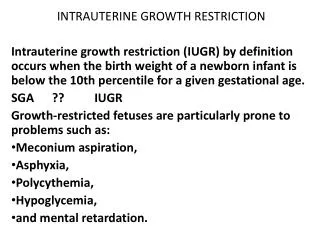
INTRAUTERINE GROWTH RESTRICTION Intrauterine growth restriction (IUGR) by definition occurs when the birth weight of a newborn infant is below the 10th percentile for a given gestational age. SGA ?? IUGR Growth-restricted fetuses are particularly prone to problems such as:
654 views • 21 slides
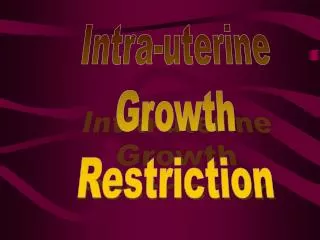
Intra-uterine Growth Restriction. Intra Uterine Growth Retardation. Intra Uterine Growth R estriction. S mall for gestational age (SGA). Foetal growth restriction. 'wasted' and 'stunted'. Definition.
723 views • 30 slides
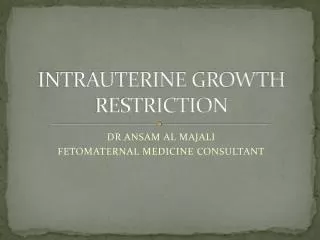
INTRAUTERINE GROWTH RESTRICTION. DR ANSAM AL MAJALI FETOMATERNAL MEDICINE CONSULTANT. Definition
477 views • 23 slides
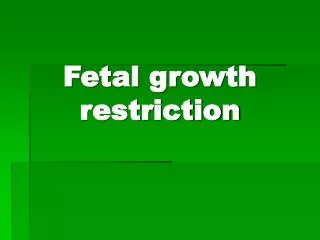
Fetal growth restriction. Fetal growth restrictions **There are wide variety of reasons why a baby may be born small including congenital anomalies , feta infections and chromosomal abnormalities .
697 views • 25 slides
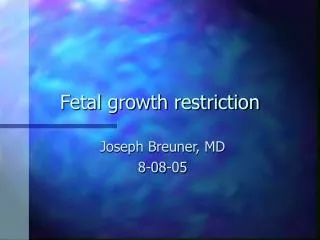
Fetal growth restriction. Joseph Breuner, MD 8-08-05. Objectives . Define risk factors Define screening Define diagnosis Define management. Take-home points. Risk factors: if positive, obtain ultrasound for growth 16-24 wks if negative, use fundal height to screen. Take-home points.
561 views • 44 slides
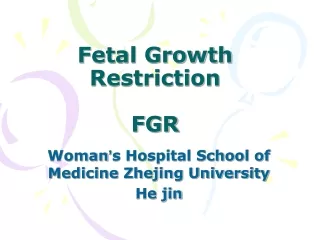
Fetal Growth Restriction FGR
Fetal Growth Restriction FGR. Woman ’ s Hospital School of Medicine Zhejing University He jin. Definition of FGR. Growth at the 10th or less percentile for weight of all fetuses at that gestational age or>37W<2500g
638 views • 57 slides
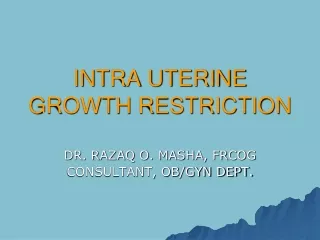
INTRA UTERINE GROWTH RESTRICTION
INTRA UTERINE GROWTH RESTRICTION. DR. RAZAQ O. MASHA, FRCOG CONSULTANT, OB/GYN DEPT. The best definition of intrauterine growth restriction (IUGR) is failure of a fetus to reach its genetic growth potential
304 views • 26 slides
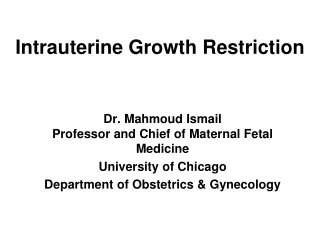
781 views • 62 slides
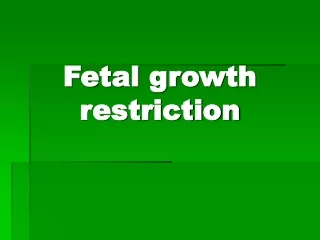
354 views • 25 slides
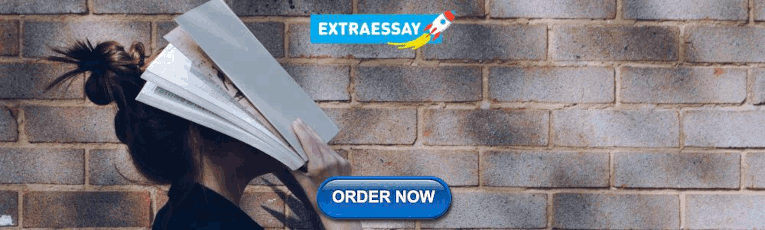
IMAGES
VIDEO
COMMENTS
3. Guidelines for data collection, analysis and presentation of fetal growth restriction. It was the consensus of the Brighton Collaboration Fetal Growth Restriction Working Group to recommend the following guidelines to enable meaningful and standardized collection, analysis, and presentation of information about FGR. However, implementation ...
ABSTRACT: Fetal growth restriction, also known as intrauterine growth restriction, is a common complication of pregnancy that has been associated with a variety of adverse perinatal outcomes. There is a lack of consensus regarding terminology, etiology, and diagnostic criteria for fetal growth restriction, with uncertainty surrounding the optimal management and timing of delivery for the ...
Fetal growth restriction, previously called intrauterine growth restriction, is a condition in which a fetus does not achieve its optimal growth potential. It impacts up to 10% of pregnancies and ...
This Clinical Opinion reviews the literature about the diagnosis, antepartum surveillance, and time of delivery of fetuses suspected to be small for gestational age or growth restricted. Several guidelines have been issued by major professional organizations, including the International Society of Ultrasound in Obstetrics and Gynecology and the Society for Maternal-Fetal Medicine. The ...
Intrauterine growth restriction (IUGR) is when the fetal weight is estimated to be below the 10th percentile for its gestational age. Causes range from chronic diseases or infections in the birthing parent to issues with the placenta and umbilical cord. Treatment includes frequent fetal monitoring and testing and, possibly, early delivery.
Introduction. Fetal growth restriction (FGR) is the condition in which a fetus does not reach its intrinsic growth potential and in which the risk of severe complications in both the short- and the long term is increased. 1-12 FGR is a common complication of pregnancy that occurs in five to ten percent of all pregnancies. 13,14 The most common pathophysiological mechanism is placental ...
Fetal growth restriction (FGR) is a condition in which an unborn baby (fetus) is smaller than expected for the number of weeks of pregnancy (gestational age). It is often described as an estimated weight less than the 10th percentile. This means that the baby weighs less than 9 out of 10 babies of the same gestational age. Newborn babies with FGR may be called "small for gestational age."
INTRODUCTION. Identification of fetal growth restriction (FGR) is an integral component of prenatal care as it is a leading cause of perinatal morbidity and mortality [].When sonographic criteria for diagnosis of FGR are met (), the obstetric provider needs to assess the severity, determine the probable cause, counsel the parents, closely monitor fetal growth and well-being for the remainder ...
The book presents the most current thinking about fetal growth restriction including: the concept of fetal growth potential which is an individualized approach for each fetus to be used as its own control; the early detection of growth restriction and transition from adaption to fetal growth pathology; the pathophysiology and
The work presented in the presentations, videos, and other content on this site ("Presentations") includes publicly available medical evidence, a consensus of medical practitioners, and/or opinions of individual practitioners that may differ from consensus opinions. ... Selective Fetal Growth Restriction (sFGR) - Current Understanding and ...
Fetal growth restriction, previously called intrauterine growth restriction, is a condition in which a fetus does not achieve its optimal growth potential. It impacts up to 10% of pregnancies
New ISUOG Practice Guidelines provide definitions of fetal growth restriction and small-for-gestational age, and describe the best possible management options based on current data and knowledge. Read the free-access ISUOG Practice Guidelines.
collection, analysis, and presentation for fetal growth restriction as an adverse event following immunization Fetuses that fail to meet their growth potential in utero are at risk for adverse antenatal and postnatal events such as stillbirth, preterm birth, and adverse neonatal and long-term health out-comes [1-5].
A fetus diagnosed to be small at ultrasound requires a structured diagnostic work-up in order to achieve an optimal ante- and perinatal management. Fetal or intrauterine growth restriction (FGR/IUGR) affects approximately 5 - 8% of all pregnancies and refers to a fetus not exploiting its genetically determined growth potential.
Fetal growth restriction (FGR) is a major cause of stillbirth, prematurity and impaired neurodevelopment. Its etiology is multifactorial, but many cases are related to impaired placental development and dysfunction, with reduced nutrient and oxygen supply. The fetus has a remarkable ability to respond to hypoxic challenges and mounts protective ...
Presentation Transcript. Fetal growth restriction Joseph Breuner, MD 8-08-05. Objectives • Define risk factors • Define screening • Define diagnosis • Define management. Take-home points • Risk factors: if positive, obtain ultrasound for growth 16-24 wks • if negative, use fundal height to screen. Take-home points • Screening: use ...
Presentation Transcript. Fetal Growth Restriction Steven R. Allen, MD Scott & White Clinic TAMU-HSC. Educational Objectives • Review epidemiology and significance of fetal growth restriction (FGR) • Know etiologies (= risk factors) • Discuss screening strategies for FGR • Evaluate the role for Doppler velocimetry in the diagnosis & mgmt ...
Defective placentation can result in fetal growth restriction and other pregnancy complications 26,27. Although details need to be elucidated, our data suggest that pre-conception paternal ...
Introduction: Violence during pregnancy (VDP) is a prevalent global issue with dire consequences for the mother and the developing fetus. These consequences include prematurity, low birthweight, and intrauterine growth restriction (IUGR), but its pathways remain elusive. This study investigated the causal pathways between VDP and IUGR using mediation analysis. Methods: A prospective population ...
Fetal growth restriction. Joseph Breuner, md March 15, 2011. Teaching points. 16 wk rule Small vs in trouble Safer out or in: <34 wks use normal UA flow by DV to continue >34 wks deliver if Maternal htn Growth arrest x 3-4 wks Bpp low (<6/8) DV UA reversed or absent. Teaching points. Download Presentation. sga parent.
Using two-dimensional ultrasound, we measured fetal adrenal diameters and adrenal/abdominal circumference (AD/AC) ratio between 25 and 37 weeks. We considered only one measurement per fetus. In 21 pregnancies we also measured NA, A, and cortisol levels in arterial and venous fetal cord blood collected at the time of delivery. Results